Interactions between white pine blister rust, bark beetles, and climate over time indicate vulnerabilities to limber pine health
- 1Forest Health Protection, Rocky Mountain Region, U.S. Department of Agriculture, Forest Service, Golden, CO, United States
- 2Rocky Mountain Research Station, U.S. Department of Agriculture, Forest Service, Fort Collins, CO, United States
- 3Department of Agricultural Biology, Colorado State University, Fort Collins, CO, United States
Introduction: Limber pine is a keystone species in the Rocky Mountains that grows on harsh, high elevation sites where few other tree species can. Recent studies suggest the species is threatened by the combined impacts of the exotic, invasive disease, white pine blister rust (WPBR), native bark beetles, and climate change. Information on changes in the health of limber pine populations and long-term impacts posed by these threats is needed to inform management efforts.
Methods: We established 106 long-term monitoring plots in 10 study areas that were surveyed three times between 2004 and 2017. We assessed site and stand factors, tree health, and regeneration over time to detect changes in limber pine abundance and health, cumulative impacts of WPBR and bark beetles, and to evaluate the drivers of WPBR occurrence and severity.
Results: Limber pine health declined significantly over the study with more than 20% of initially live limber pine trees dead by the last measurement cycle, primarily due to WPBR and bark beetles. While some recruitment occurred, mortality rates greatly outpaced recruitment of ingrowth. Disease incidence and how it changed over time was variable, but disease severity increased substantially overall and in all study areas. Limber pine regeneration was low or absent in most sites and mortality caused by WPBR increased significantly. We found strong relationships between WPBR and aridity. Trees in habitats with high vapor pressure deficit were less likely to be infected with WPBR, but trees that were already infected were more likely to develop severe symptoms and die. Longer growing seasons increased the likelihood of WPBR presence and mortality. Growing season length and vapor pressure deficit tended to increase over the study, suggesting that climate change may exacerbate disease impacts.
Discussion: Declining health of limber pine coupled with high mortality rates, increasing disease severity, and low levels of natural regeneration indicate successful recovery may not occur in some locations without management intervention. Proactive management strategies to reduce insect and disease impacts and promote stand recovery and resilience should be pursued in remaining, healthy limber pine ecosystems.
1. Introduction
Limber pine (Pinus flexilis) is an ecologically and culturally important tree species that occurs throughout western North America spanning a vast latitudinal (34°–54° N) and elevational (870–3,810 m) range (Steele, 1990; Figure 1). Not only is limber pine an important component of the forested ecosystems it inhabits, as a keystone species it has a disproportionately large influence on ecosystem functions relative to its abundance (Steele, 1990; Schoettle et al., 2022a). Limber pines provide habitat and a food source for wildlife, regulate snow retention and runoff, facilitate the establishment of late successional tree species at treeline, and maintain cover on harsh, rugged sites where little else can grow (Schoettle, 2004). Limber pines are threatened by the combined impacts of climate change, bark beetles, and the exotic, invasive disease, white pine blister rust (WPBR) (Burns et al., 2011; Smith et al., 2013a; Kearns et al., 2014; Cleaver et al., 2015; Schoettle et al., 2022a).
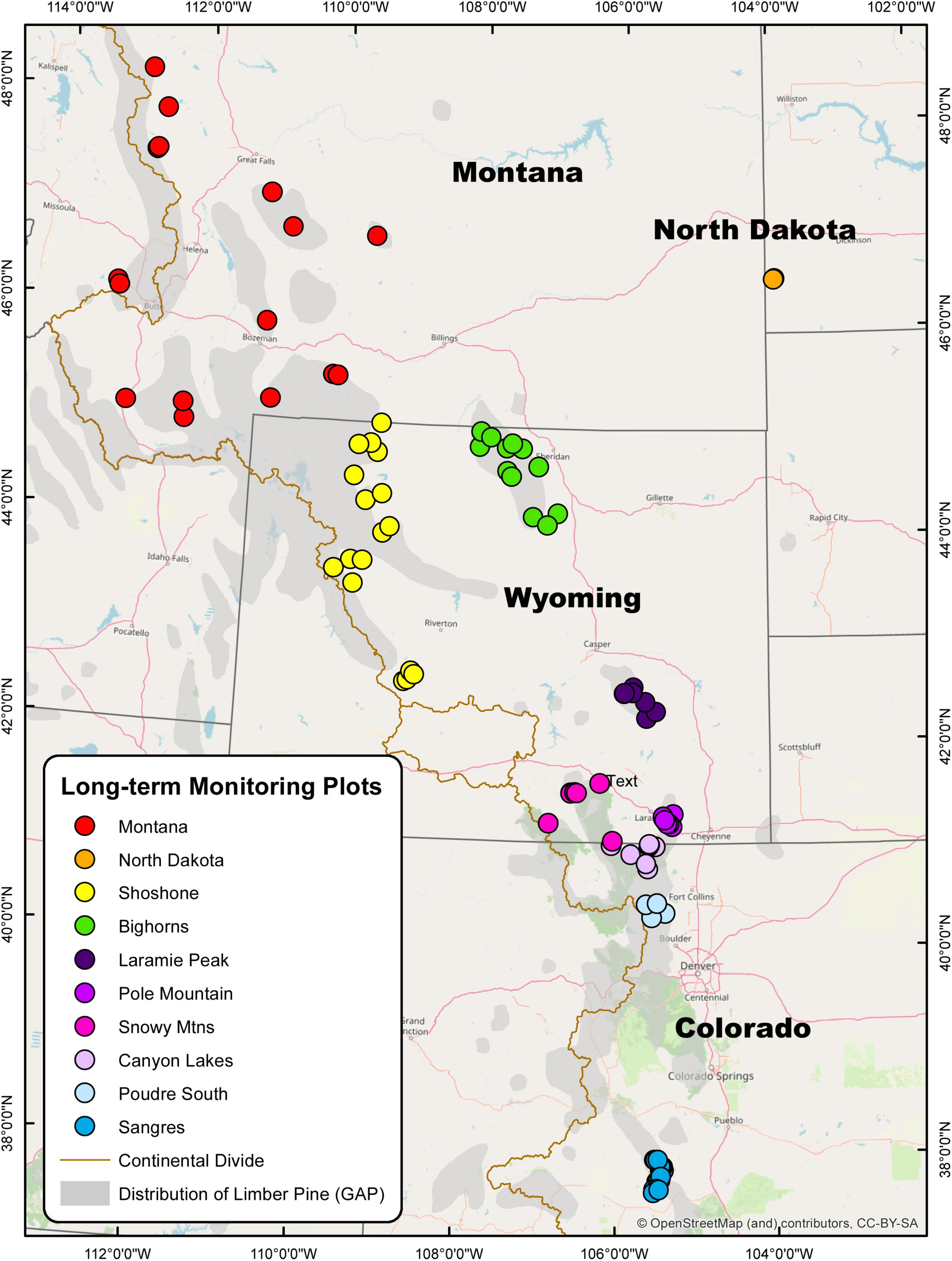
Figure 1. Map of 106 long-term limber pine health monitoring plots measured three times between 2004–2007 and 2016–2017 in the U.S. Rocky Mountains. The 10 study areas are denoted by color and include Montana (16), North Dakota (2), Shoshone (17), Bighorns (12), Laramie Peak (7), Pole Mountain (8), Snowy Mountains (7), Canyon Lakes (8), Poudre South (4), and Sangre de Cristos (25). Limber pine distribution (denoted in gray) was gathered from the USGS Gap Analysis Project (GAP).
Recent studies indicate limber pine health in the Rocky Mountains is declining (Jackson et al., 2010; Smith et al., 2013a; Cleaver et al., 2015, 2022; Shanahan et al., 2019) and mortality significantly exceeds growth (Goeking and Windmuller-Campione, 2021). Climate models predict that limber pine will be forced to higher elevations (Monahan et al., 2013) and the USDA Forest Service National Insect and Disease Risk Map predicts a 44% reduction in limber pine basal area by 2027 due to combined effects of WPBR, bark beetles, and dwarf mistletoe (Krist et al., 2014). Concerns over the status of the species have led limber pine to be listed as a “Species of Local Concern” on the Black Hills National Forest, a “Species of Management Concern” in Rocky Mountain National Park, and a “BLM Sensitive Species” in Wyoming. The listing of limber pine as Endangered under the Species at Risk Act in Canada is pending (COSEWIC, 2014). The Committee on the Status of Endangered Wildlife in Canada (COSEWIC) concluded “this tree species is imminently and severely threatened throughout its Canadian range by white pine blister rust (an introduced pathogen), mountain pine beetle, and climate change.”
The fungal pathogen that causes WPBR, Cronartium ribicola, was inadvertently introduced into western North America in the early 20th century (Geils et al., 2010). All nine North American white pine species are susceptible to the rust. WPBR was first reported in natural limber pine stands in southern Idaho in 1945 (Krebill, 1964) and has since slowly spread throughout a large portion of limber pine’s range, now occurring in areas once thought to be too inhospitable for the pathogen to flourish (Blodgett and Sullivan, 2004; Burns, 2006; Vogler et al., 2017a,b; Jacobi et al., 2018a; Schoettle et al., 2018). The only states where WPBR has not been detected in limber pine to date include Utah, Oregon, and California (Kliejunas and Dunlap, 2007; Maloney, 2011; Dunlap, 2012; Vogler et al., 2017a). The relatively recent movement of the disease into limber pine forests of Colorado and portions of southern Wyoming coupled with the rising bark beetle epidemic, raise questions as to the future health of white pines in high-elevation forests of the U.S. Rocky Mountains.
Cronartium ribicola is a macrocyclic, heteroecious rust fungus and therefore requires an alternate host (Ribes, Pedicularis, or Castilleja species) to complete its life cycle. Sporulation, dispersal, and infection occur only when specific temperature and humidity requirements are met (McDonald and Hoff, 2001). “Wave years” with heavy pine infection occur during years when microclimatic conditions are suitable for C. ribicola infections. Unlike the Northern Rocky Mountains, wave years are likely infrequent in the warmer and drier ecosystems of the Southern Rocky Mountains leading to slower disease spread and intensification (Jacobi et al., 2018a). Spores produced on pines (aeciospores) in late spring/early summer infect alternate hosts; there is no pine-to-pine transmission. Aeciospores are thick-walled and hardy and can travel long distances (>100 km) in the wind. Basidiospores produced on alternate hosts in late summer/early fall infect pines. They are fragile and only travel short distances (1 km) in the wind. Following infection through needle stomata, fungal mycelium grows into the phloem eventually causing a canker. If an infection occurs close to the main stem, it will eventually grow into it and girdle the stem, killing the portion of the tree above the canker. Impacts on pines include branch death and topkill which reduce cone-production, and whole-tree mortality. The time it takes from infection to mortality depends on the location of the infection and tree size; small trees are killed rapidly (Geils et al., 2010).
Bark beetles may interact with WPBR to exacerbate declining health of limber pine. Mountain pine beetle (Dendroctonus ponderosae; MPB) is an aggressive, destructive bark beetle native to western North America. Nearly all western pine species are hosts, but lodgepole (Pinus contorta), ponderosa (P. ponderosa), whitebark (P. albicaulis), and limber pine are the most common hosts in the Rocky Mountains (Furniss, 1977; Alfaro et al., 2003; Gibson, 2003; Brown and Schoettle, 2008; Gibson et al., 2008). The most recent outbreak (roughly 1998–2013) particularly affected limber pine, a favored host (Langor, 1989; Cerezke, 1995), with concentrated mortality occurring on over 500,000 ha in the Rocky Mountain Region and coinciding with severe drought, warm temperatures, and mild winters (Logan and Powell, 2001; Taylor et al., 2006; Vorster et al., 2017). As the MPB epidemic collapsed in northern Colorado and southern Wyoming, populations of Ips woodi increased, attacking, and killing limber pines in the absence of MPB (Witcosky, 2017). Ips activity continued for several years adding to the total losses of limber pine over the course of the epidemic.
The ability of limber pine to successfully regenerate is complicated by a variety of factors such as WPBR, its relatively slow ability to recover following natural disturbance (Schoettle, 2004; Coop and Schoettle, 2009), and climate change. WPBR quickly girdles young trees and cone-bearing branches on mature trees (Geils et al., 2010). Bark beetles can indiscriminately kill mature WPBR-resistant trees, reducing the favorable gene pool (Schwandt, 2006). Furthermore, evidence suggests that climates are becoming less hospitable for white pines (Rehfeldt et al., 2008; Larson, 2011; Malone et al., 2018; Millar et al., 2018), further exacerbating the ability for these species to recover. Knowledge of the current health status of limber pine and how stands are impacted by these damage agents over time will provide critical information to inform management and guide recovery efforts (Schoettle et al., 2022a).
Recent studies have surveyed limber pine health in a single time point (Burns, 2006; Kearns and Jacobi, 2007; Kliejunas and Dunlap, 2007; Burns et al., 2011; Klutsch et al., 2011; Maloney, 2011; Dunlap, 2012; Cleaver et al., 2015, 2017b,2022; Shanahan et al., 2019) as well as in multiple time points (Smith et al., 2013a). In single time-point surveys, incidence and mortality rates show great variability between and even within mountain ranges (Burns, 2006; Kearns and Jacobi, 2007; Burns et al., 2011; Cleaver et al., 2015), demonstrating the complexity of forest health trends in the limber pine-WPBR pathosystem. Sites (not permanently monumented) in Wyoming and Colorado first measured by Kearns and Jacobi (2007) in 2002–2004 and remeasured by Cleaver et al. (2015) in 2011–2012 found WPBR incidence increased 6% (from 20 to 26%) and bark beetle mortality increased 17%. Smith et al. (2013a) surveyed limber pine throughout its range in the Canadian Rocky Mountains over 6 years from 2003 to 2009, with some sites surveyed over 13 years (1996–2009). They found incidence increased 10% and the disease continued to spread into new locations. Limber pines at the southernmost edge of their study, near the U.S.-Canada border, showed the highest WPBR infection and mortality rates. Results suggested long-term persistence of limber pine in some sites is in jeopardy.
Long-term surveys of permanent plots provide valuable insights into disease spread and development, which can be especially important in forested ecosystems, as changes to tree health may span many years (Cleaver et al., 2017a; Schoettle et al., 2019a,b). We assessed permanent plots throughout the U.S. Rocky Mountains and North Dakota over time to measure changes in health status and stand dynamics in limber pine populations. The objectives were to: (1) evaluate changes in limber pine abundance and health, (2) assess long-term, cumulative impacts of WPBR and bark beetles, and (3) evaluate site, stand, and environmental drivers of WPBR occurrence and severity at the tree and plot level. Results of this long-term study provide insight into temporal trends of the biotic agents threatening ecologically important limber pines to inform and guide management efforts.
2. Materials and methods
2.1. Study areas
The survey area extended through most of the distribution of limber pine in the U.S. Rocky Mountains and North Dakota. Permanent plots, ca. 106, were established within 10 study areas during the 2004 (Sangre de Cristo Mountains), 2006 (Poudre Canyon South, Canyon Lakes, Snowy Mountains, Pole Mountain, Laramie Peak, Bighorn NF, Shoshone NF), and 2007 (North Dakota, Montana) field seasons (Figure 1). Subsequent assessments took place from 2011 to 2014 and again in 2016 to 2017 (Supplementary Table 1). The northernmost plot was located on the Blackfeet Indian Reservation, Montana (48°15′7″ N, 112°48′2″ W) and the southernmost was on the Rio Grande National Forest, Colorado (37°36′41″ N, 105°31′48″ W). Two plots occurred on the west side of the Continental Divide, west of Butte, Montana, and the easternmost plots were in the badlands of western North Dakota. Plot locations were selected based on vegetation layers, limber pine composition >20% in previous surveys, and suggestions from local land managers. Plot locations were randomized across elevations, aspects, slope positions, WPBR incidence (where available), and stand species compositions.
2.2. Plot design
Plots were established as belt transects following methods adapted from the Whitebark Pine Ecosystem Foundation (Tomback et al., 2005). Each plot (60 × 15 m, 0.09 ha) was divided into three 20 × 15 m sections, except in the Sangre de Cristo Mountains where plot dimensions varied to include approximately 30 live white pines >1.37 m tall; but on average were 60 × 15 m (Burns et al., 2011). At the center point of each section, a fixed area circular subplot (0.004 ha, 3.6 m radius) was established to quantify ground cover, understory vegetation, and regeneration (stems <1.37 m tall). Plots were monumented with a labeled rebar stake (or PVC pipe) at the center point of each section and at the plot start and end points.
2.3. Survey methods
2.3.1. Site measurements
Site data collected included location (latitude/longitude), aspect (degrees), slope (percent), elevation (meters), slope position (summit, ridgetop, or plateau; shoulder; backslope; footslope; toeslope; valley bottom), stand structure (closed canopy single story, closed canopy multi-storied, open canopy scattered individuals, open canopy scattered clumps, mosaic), disturbance history (e.g., fire scars, tree cutting, mining activity, avalanche, and rock slides), and presence/absence of WPBR alternate hosts (Ribes, Castilleja, and Pedicularis spp.).
2.3.2. Trees >1.37 m in height
All trees (white pines only in the Sangre de Cristo Mountains) greater than 1.37 m tall were tagged and the following data were collected: species, diameter at breast height (dbh), health status (healthy: <15% damage to crown/stem; declining: 16–50% damage to crown/stem; dying: >50% damage to crown/stem; recent dead: no green needles, red needles/fine twigs present; old dead: no fine twigs, no needles), crown class (open grown, dominant, codominant, intermediate, overtopped, or krummholz), and damage agents and their severities. Trees growing in clumps were considered individual stems if they were distinct at breast height (1.37 m). For white pines, additional information was collected including live crown ratio (the percent of total tree height that supports live foliage), canopy kill (visual estimate of the percentage of the canopy that was recently killed not including old dead branches lacking fine twigs or with <50% bark intact), presence or absence of live cones, and measurements to characterize WPBR presence and severity (see section “2.3.3. White pine blister rust assessments”). Trees that grew taller than 1.37 m during a measurement cycle were included as “ingrowth,” tagged, and the above metrics were recorded. Cause of death was attributed to WPBR when an obvious extensive, lethal stem canker was present (regardless of beetle activity). Alternately, cause of death was attributed to bark beetles if the tree was mass attacked and did not have an extensive, lethal canker (otherwise, regardless of WPBR status). Year of attack for bark beetle-killed trees was estimated based on degradation classes of needles and fine branches as described by Klutsch et al. (2009). In the initial measurements, trees classified as “old dead” were not evaluated for damage or cause of death, as many were too degraded to accurately determine this information.
Species composition was assessed by categorizing stems as either limber pine or falling into one of the following categories: “Other Pines”: lodgepole and ponderosa pine; “Other White Pines”: whitebark and Rocky Mountain bristlecone (Pinus aristata) pine; “Spruce-Fir”: Douglas-fir (Pseudotsuga menziesii), subalpine fir (Abies lasiocarpa), white fir (Abies concolor), and Engelmann spruce (Picea engelmannii); or “Other Spp.”: Rocky Mountain juniper (Juniperus scopulorum) and quaking aspen (Populus tremuloides). In the Sangre de Cristo Mountains, a variable radius plot was established at the center point of the beginning, center, and end of each plot to collect data on stand composition including species, diameter class for trees taller than 1.37 m (0.1–12.6, 12.7–30.5, >30.5 cm dbh), and health status (see above) for all “in” trees.
Annualized mortality rates (M) were calculated as M = 1 − (Ts / T1)1/Δt where Ts represents the number of live limber pine stems (>1.37 m tall) assessed during the first measurement cycle (2004/2006/2007) that were still alive in the final measurement cycle (2016/2017), T1 represents the number of live limber pine stems (>1.37 m tall) assessed during the first measurement cycle, and Δt represents the number of growing seasons between each individual plot’s first and final measurements (Dudney et al., 2020). Similarly, ingrowth recruitment rates (R) were calculated as R = 1 − (Ts / T3)1/Δt where Ts represents the number of live limber pine stems (>1.37 m tall) assessed during the first measurement cycle (2004/2006/2007) that were still alive in the final measurement cycle (2016/2017), T3 represents the number of live limber pine stems (>1.37 m tall) assessed during the final measurement cycle, and Δt represents the time interval (Dudney et al., 2020).
2.3.3. White pine blister rust assessments
Number of branch and stem cankers per crown and stem third were tallied for each infected white pine. This was done by visually dividing the entire length of the crown (using a planar method) and stem into thirds. In the absence of aecia or pycnia, at least three of the following five indicators needed to be present: roughbark, flagging, gnawing, sap production, and/or swelling. Stem cankers included all cankers on the main stem or within 15 cm of it. Branch canker lengths (horizontal extent of cankered bark, no measurements of radial canker extent were taken) were visually estimated for up to six branch cankers per crown third. These data were used as a surrogate for canker age allowing us to roughly estimate the year WPBR became established on a site and/or the frequency of infection events (Kearns et al., 2009). Cankers were put into 12-cm size classes that represented average annual canker growth (e.g., size 1 = >0–12 cm, size 2 = 12.1–24 cm, size 3 = 24.1–36 cm, etc.). Frequency of infection events was based on the number of canker size classes that occurred within a subregion. For example, if there were cankers in each of the 10 first size classes, we inferred infections occurred every year for the previous 10 years.
White pine blister rust disease severity was calculated for each tree based on cumulative crown and stem damage (Six and Newcomb, 2005). Crowns and stems were divided into thirds and evaluated based on the percentage of branches and bole circumference affected by cankers. For each crown and stem third, a score of 0 was assigned to 0% affected, 1 for <25% affected, 2 for 25–50% affected, and 3 for >50% affected. The 6 total scores per tree (3 crown and 3 stem) were summed to get the tree’s severity rating. The maximum severity score possible for a tree was 18, however scores above 14 are unlikely as few trees survive with a score >12 (Six and Newcomb, 2005). Stand severity was characterized by calculating a mean severity score for all infected trees in the plot. Stand descriptors for rust infection included scores which ranged from 1 to 4.9 (light infection), 5 to 8.9 (moderate infection), and 9+ (heavily infected). Disease severity data were not collected in the Sangre de Cristo Mountains.
2.3.4. Regeneration
All regenerating tree species <1.37 m tall were evaluated within the three subplots (0.004 ha, 3.6 m radius). Data collected included species; height class (<25 cm or 25–137 cm); WPBR presence/absence; and cause of death (WPBR/not WPBR) for white pine species (see section “2.3.2. Trees >1.37 m in height”). Beginning in the 2011–2014 measurement cycle and onward, all white pine regeneration within the entire plot (60 m × 15 m, 0.09 ha) were tallied by species, with observations of WPBR presence/absence, and cause of death (WPBR/not WPBR). Regenerating species other than limber pine were only characterized from subplot data.
Additional data to assess the presence or absence of microsite conditions available for successful regeneration were collected in subplots. This included estimating percent ground cover of lichen/moss, rock, bare soil, litter, vegetation (shrubs and forbs), and tree stems/downed logs. The three most common shrub species were also noted.
2.4. Meteorological data
Meteorological data were summarized for the 4- and 15-year periods prior to the survey year for each plot per measurement cycle to assess their influence on C. ribicola occurrence, incidence, and severity. Information is lacking on the incubation period of C. ribicola on limber pine in the Rocky Mountains. The 4-year cycles were used because early research in western white pine (Pinus monticola) indicates that while the timing varies by factors such as tree size and environmental conditions, 4-years is roughly the maximum length of time that it takes from infection to the appearance of obvious signs and symptoms of disease in the field (Lachmund, 1933).
Mean and total snow water equivalent were summarized for each 4- and 15-year period and maximum temperature, minimum temperature, average temperature, precipitation, and growing degree days (>5°C) were summarized for August and September (AS), the time when pine infection typically occurs; May to September (MS), the biological window when most C. ribicola sporulation occurs on pines and alternate hosts; and an annual average for the 4- and 15-year period using Daymet’s 1-km daily surface weather grids (Thornton et al., 2016). Relative humidity (AS, MS, average annual) was derived from the Daymet dataset following the methods of Allen et al. (2006). Maximum and minimum vapor pressure deficit (daily values averaged for AS, MS, and the 4-year period) were obtained from PRISM Climate Group’s 4-km recent year’s climate data grids (PRISM Climate Group, Oregon State University).1
2.5. Data analyses
Statistical calculations were completed in SAS 9.4, JMP v14, or R (version 4.0.3; R Core Team, 2015). WPBR incidence values were estimated for each plot by calculating the number of live, infected trees out of total live limber pine surveyed. WPBR mortality was calculated as the number of limber pines killed by C. ribicola out of the total number (live and dead) limber pine. Mean incidence and mortality were determined by study area and overall using a generalized linear mixed model, PROC GLIMMIX, procedure in GLMM mode with plot location as random effect and year as fixed effect. Stand means were assessed for significant change (P < 0.05) by comparing the first measurement to the last measurement using t-tests in JMP or PROC TTEST option “paired” on count data (not percentages) in SAS. Standard error was calculated for the change between two means, pooling variance across measurements within a study area.
To evaluate the influence of site and stand factors and meteorological variables on the occurrence and severity of WPBR, generalized linear and logistic mixed-effects models were fit. Prior to model fitting, all climate variables were centered and scaled to enable comparison of model coefficients for variables measured on different scales (relative humidity, precipitation). Evaluation of meteorological data revealed summarization for the 4-years prior to each plot’s measurement year provided the strongest correlation with WPBR parameters and thus only the 4-years data were used for modeling. All of the tree, plot, and climatic variables were evaluated for collinearity prior to model fitting, with variables exceeding 0.7 correlation removed. The model of WPBR occurrence was structured as a logistic regression from a binomial distribution with logit-link, where the presence of WPBR on a plot was denoted as a 1 and absence as a 0. The occurrence model treated plot and measurement period as random-effects, while evaluating the temporally dynamic fixed-effects. For the multiple linear models of WPBR severity change, it was necessary to use a Gamma distribution with log-link to account for the non-negative distribution of the response variable. In the severity model, plot was treated as a random-effect and years since the first measurement cycle at each plot (0–13 years) was added as a fixed-effect. The R statistical program was used for all modeling and significance testing using the lme4, lmerTest, and sjstats packages (Bates et al., 2014; Kuznetsova et al., 2017; Lüdecke et al., 2020). Parameters were backward eliminated from the models until the Akaike information criterion (AIC) could not be reduced further.
3. Results
3.1. Site characteristics
Plots were distributed across a variety of aspects, slopes, and slope positions and were located between 884–3,119 m (2,900–10,243 ft) elevation (Supplementary Table 2). When the study began, 7,174 (6,884 live) standing trees were assessed including 3,863 (3,623 live) limber pines (Supplementary Table 2). During the final assessment, plots contained 8,129 (6,461 live) standing trees, of which 4,176 (3,141 live) were limber pine (Supplementary Table 2). Plots had 34 (range 1–169) and 30 (range 1–162) live, limber pines on average at the start (2004–2007) and end (2016–2017) of the study, respectively. The percentage of plots with Ribes spp. present increased from 54 to 66% over the study. Other alternate hosts (Castilleja spp. and/or Pedicularis spp.) were present in 37% of plots during the final measurement cycle (data not collected in earlier measurements) (Supplementary Table 1).
3.2. Stand structure, species composition, and demographic rates
Mean dbh was 12.7 and 13.0 cm for live limber pines and 15.2 and 14.5 cm for dead limber pines, at the start and end of the study, respectively. At the beginning of the study, most recent mortality was mature trees (>12.7 cm dbh) and was attributed to bark beetles (Table 1 and Supplementary Table 6). By the end of the study, most recent mortality was small trees (0.1–12.7 cm dbh) and was attributed to WPBR. Despite high levels of mortality, surveyed limber pine populations displayed a reverse-J size-class distribution throughout the study. This is typical in stable uneven-age stands, because the density of small trees is much greater than the density of large trees (O’Hara, 2002). While the proportion of limber pine stems to other tree species decreased by 4% (from 53 to 49%, Supplementary Table 2), the proportion that other co-occurring tree species contributed to species composition did not change significantly from start to end. After limber pine, spruce-fir and other species (Rocky Mountain juniper and quaking aspen) contributed the most to species composition (both contributed 16 and 18% to live stems at the start and end respectively) followed by other pines (13 to 11% of live stems, respectively), and other white pines (3 to 4% of live stems, respectively). The proportion of live limber stems declined slightly, though not significantly, in all study areas, except Laramie Peak and North Dakota (Supplementary Table 2).
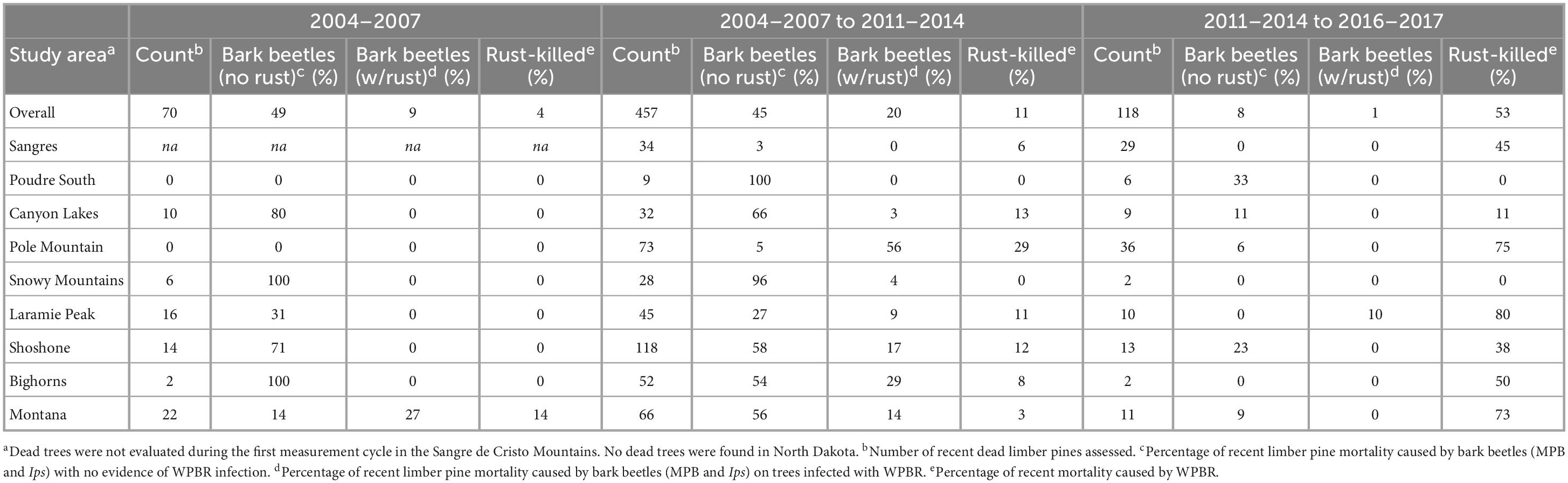
Table 1. Causes of mortality for recently killed trees (>1.37 m tall) in each of the three measurement cycles.
Overall, average live tree density (stems ha–1) declined significantly overall for all tree species over the study period (−55 ± 25 stems ha–1, P = 0.03), and this was most pronounced in the Pole Mountain study area (−274 ± 84 stems ha–1, P = 0.01; Supplementary Table 4a). However, while not significant, increases in aspen (Poudre South), spruce-fir (Snowy Mountain and Bighorns), and other pines (Bighorns) were observed (Supplementary Table 2). Live limber pine density also decreased significantly overall from 383 stems ha–1 in 2004–2007 to 333 stems ha–1 in 2016–2017 (−50 ± 10 stems ha–1, P < 0.001). Decreases were greatest on Pole Mountain (−139 ± 47 stems ha–1, P = 0.02), Shoshone NF (−99 ± 30 stems ha–1, P = 0.02), and Montana (−66 ± 26 stems ha–1, P = 0.02). Similarly, live limber pine basal area decreased significantly overall (−1.1 ± 0.2 m2 ha–1, P = 0.01; Supplementary Table 4b), with the most pronounced reduction at the Shoshone NF sites (−3.6 ± 1.6 m2 ha–1, P = 0.03). Density of live limber pine greater than 1.37 m tall in the small (<12.7 cm dbh) and medium (12.7–30.5 cm dbh) diameter classes decreased significantly while density in the large (>30.5 cm dbh) diameter class remained stable over the course of the study (Supplementary Table 5a).
The annualized mortality rate for limber pine was about 4%/year overall and ranged from <1%/year in the Sangre de Cristo Mountains to 8%/year in Montana (Figure 2). Ingrowth recruitment rates were lower than mortality rates overall (∼1.25%) and in every study area. Ingrowth recruitment rates also had a narrower range than mortality rates from the lowest on Pole Mountain at <1% to the greatest on the Shoshone NF at ∼2%.
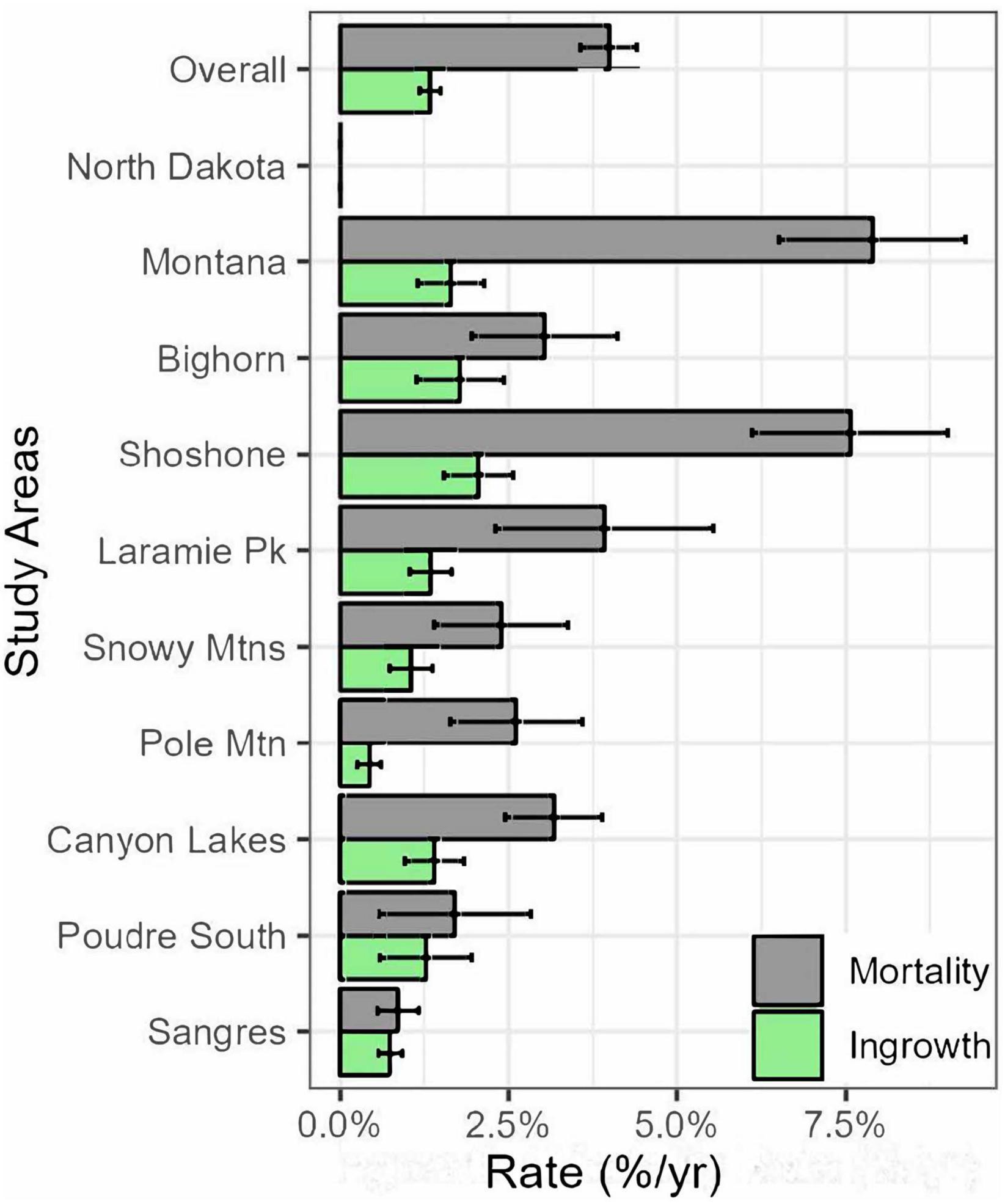
Figure 2. Annual mortality rate of trees >1.37 m tall from all causes (gray bars) and ingrowth recruitment rate (trees that grew taller than 1.37 m after the first measurement cycle; green bars) for limber pines overall and by study area from 2004–2007 to 2016–2017. Error bars indicate 95% confidence intervals.
3.3. Limber pine health status
During the first measurement cycle, 81% of limber pines >1.37 m tall were classified as healthy, 14% were declining or dying, and 5% were dead. By the end of the study, only 50% were classified as healthy, while 25% were declining or dying and 25% were dead (Figure 3). WPBR was the most common damage agent observed and was detected on 52 and 50% of live, declining/dying trees at the start and end of the study respectively. Other damage agents noted on declining trees included bark beetles (3 and 15%), twig beetles (50 and 9%), animal damage (10 and 1%), and dwarf mistletoe (4 and 2%).
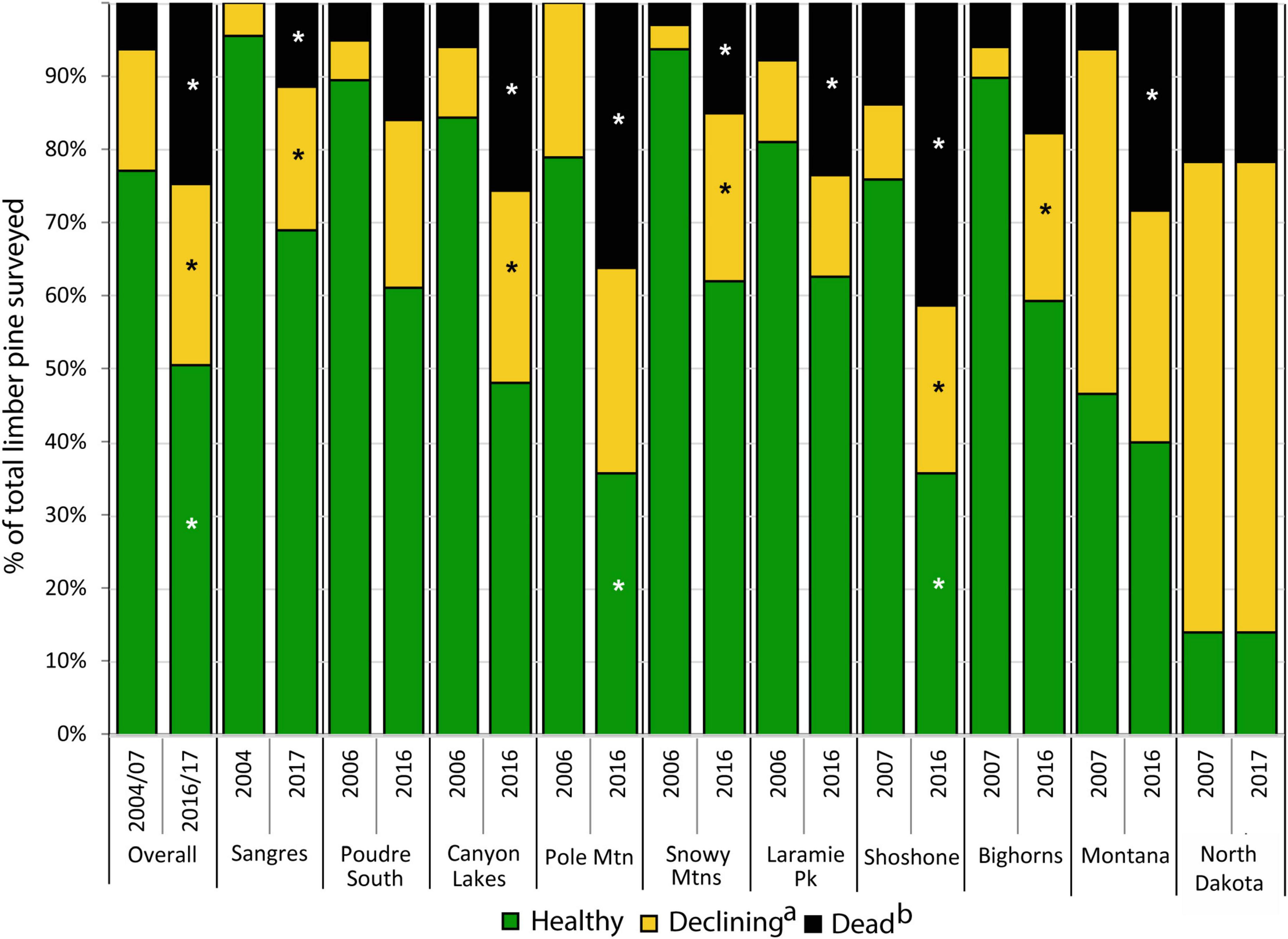
Figure 3. Changes in the health status of limber pine trees (>1.37 m tall) from 2004–2007 to 2016–2017 overall and within 10 study areas (a) includes all trees classified as declining or dying; (b) includes all trees classified as recent dead and old dead. Dead trees not measured in the Sangre de Cristos in 2004–2007. Asterisks represent significant change (P < 0.05) using paired t-test from 2004–2007 to 2016–2017 within study areas.
3.4. Limber pine mortality and causes
During the first measurement, 6% of all limber pines were standing dead (Figures 3, 4). Of the 70 recently killed trees assessed at the start of the study (cause of death not evaluated for old dead trees), 49% were killed by bark beetles with no evidence of WPBR, 9% were killed by bark beetles and were infected with WPBR, and 4% were killed by WPBR (Table 1). At that time, bark beetle mortality was detected in all study areas except Poudre South, Pole Mountain, and North Dakota. Mortality attributed to WPBR was only detected in Montana at the start of the study (Table 1). Mortality increased significantly overall and in most study areas from start to end (Figure 3) and the percentage of dead trees that were infected with WPBR increased significantly overall, and in most study areas (Figure 4; Pole Mountain, Laramie Peak, Shoshone, Bighorn, and Montana; data unavailable for Sangre de Cristos).
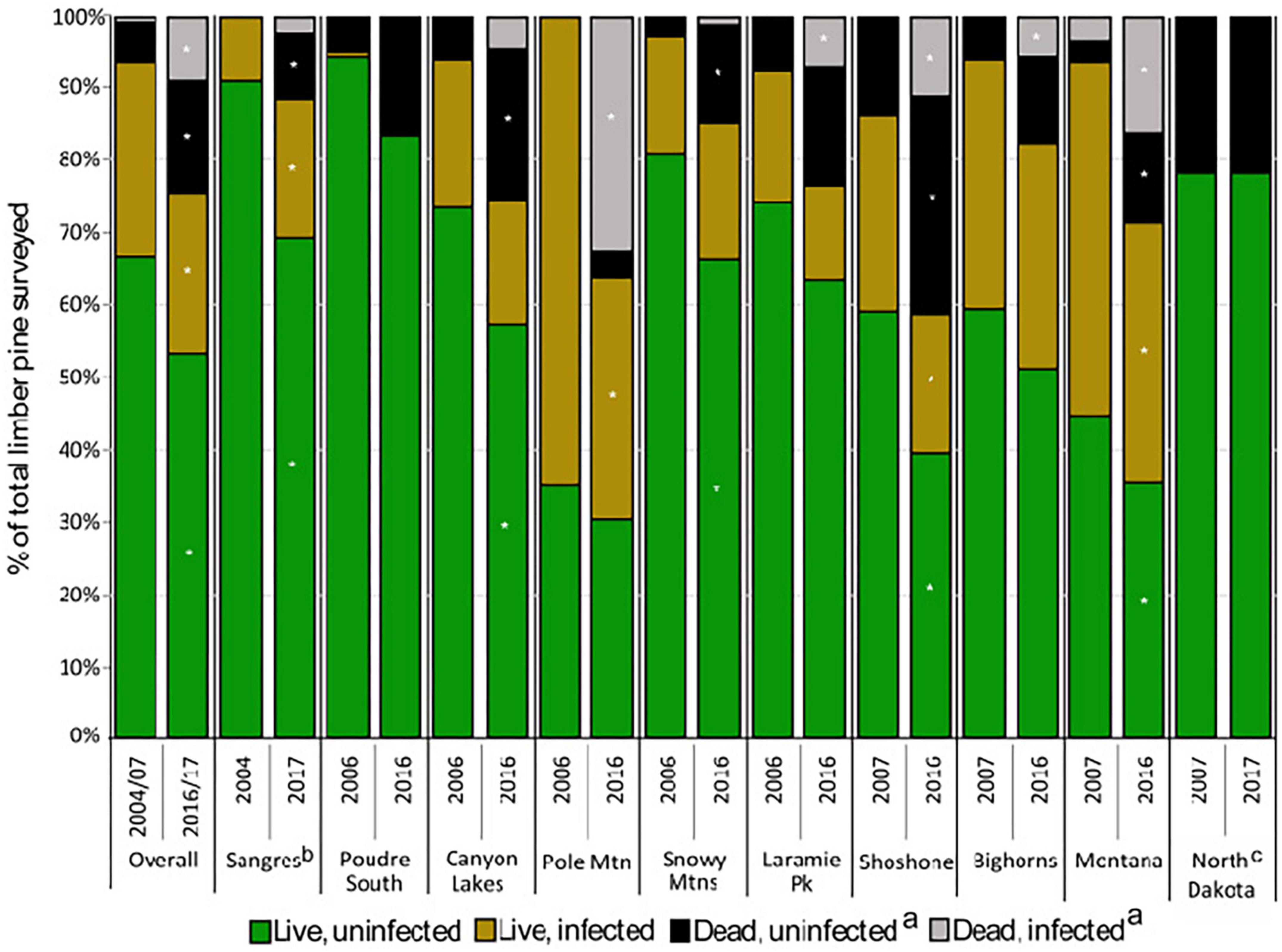
Figure 4. Status of limber pine (>1.37 m tall) and white pine blister rust infection (2004–2007 to 2016–2017) (a) dead from any cause; (b) dead trees were not recorded in the Sangres in 2004–2007; (c) rust was not detected in ND. Asterisks represent significant change (P < 0.05) using paired t-test from 2004–2007 to 2016–2017 within study areas.
By the end of the study, 25% of limber pines were standing dead, this ranged from 12% in the Sangre de Cristo Mountains to 41% on the Shoshone NF (Figures 3, 4). Further, 5.1% of all initially living trees assessed were killed by WPBR over the study (Table 2). Of the recently killed trees assessed in the second measurement cycle (2011–2014, 457 trees), 45% were killed by bark beetles and had no evidence of WPBR, 20% were killed by bark beetles and had WPBR, and 11% were killed by WPBR (Table 1). Mortality attributed to bark beetles declined sharply between 2009 and 2013 and has remained at endemic levels since. Most (53%) of the recently killed trees assessed during the final measurement cycle (2016–2017, 118 trees) were killed by WPBR while only 9% were associated with bark beetles (Table 1). Cause of death could not be determined for most of the remaining recent dead trees. A small portion were killed by other factors including twig beetles (Pityophthorus and/or Pityogenes spp.), fire, limber pine dwarf mistletoe (Arceuthobium cyanocarpum), competition, and physical effects such as wind or lightning. Mortality caused by WPBR was observed in all study areas except Snowy Mountains, Poudre Canyon South, and North Dakota. Bark beetle mortality was evident in all study areas except North Dakota but was very low in the Sangre de Cristo Mountains (<1% stems killed). Overall, more than 30% of trees killed by bark beetles were also infected with WPBR. On Pole Mountain, 84% of trees killed by bark beetles were also infected with WPBR.
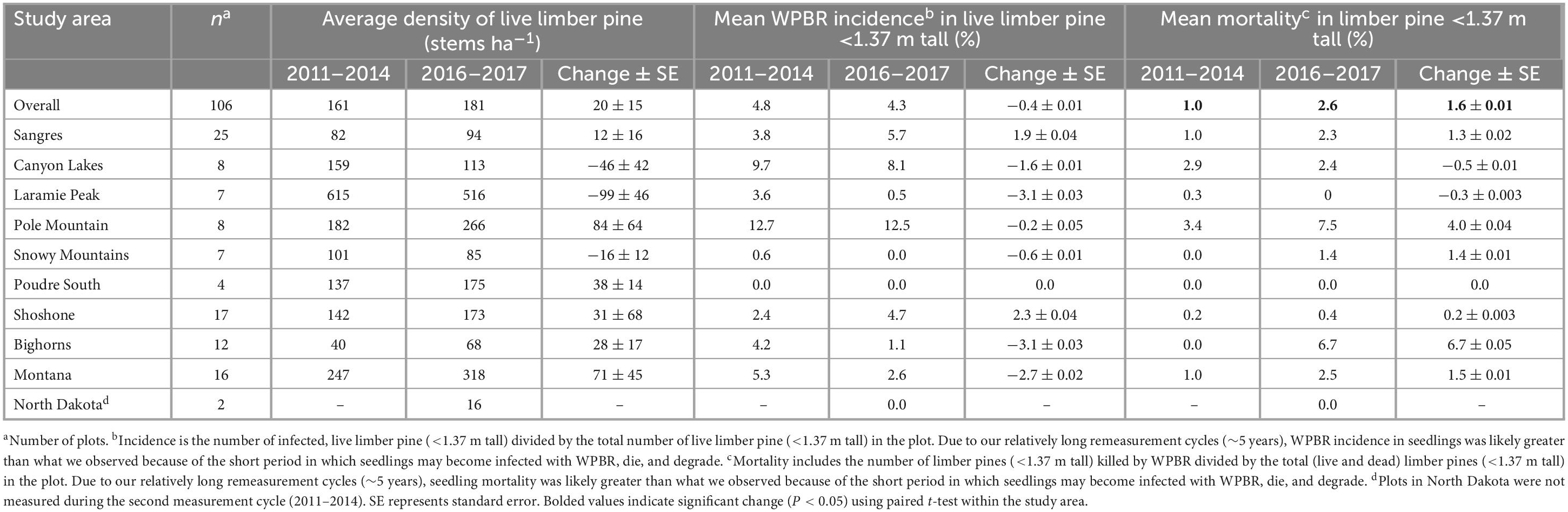
Table 2. Density of limber pine regeneration (<1.37 m tall) and WPBR incidence and mortality (2011–2013 to 2016–2017, whole plot counts of limber pine regeneration not collected in 2004–2007).
Most bark beetle-killed trees (83%) were medium or large (≥12.7 cm dbh) while most WPBR-killed trees (84%) were small (<12.7 cm dbh) in size (Supplementary Table 6). The bark beetle mortality rate was greatest on the Shoshone NF (17%) and WPBR mortality rate was greatest on Pole Mountain (20%) (Supplementary Table 6).
3.5. Incidence and severity of white pine blister rust
White pine blister rust was found in all study areas except North Dakota and on 74 and 77% of all plots both at the start and end of the study, respectively (Supplementary Table 2). Over the study, six previously disease-free sites gained WPBR, 49% of plots gained more infected trees, and 5.9% of trees that were disease-free at the start became infected by the end (Table 3). The proportion of initially disease-free trees (>1.37 m tall) that became infected was highest in the Sangre de Cristo Mountains (13.6%) and lowest in the Canyon Lakes (3.1%) study areas. No new infected trees were detected in Poudre Canyon South. Further, of trees that grew taller than 1.37 m after the first measurement cycle (“ingrowth”), 7.1% were infected by the final measurement cycle; this ranged from 2.6% on the Bighorn NF to 14.1% in Montana. Overall, 65.2% of limber pines that were alive and disease-free at the start of the study (i.e., not including ingrowth) remained disease-free at the end. The lowest proportion of limber pines that remained disease-free occurred on Pole Mountain, where only 30.8% never showed any signs or symptoms of disease (Table 3). Nearly 15% of trees that were infected during the first measurement cycle were killed by rust impacts by the last measurement cycle (range 4–29%).
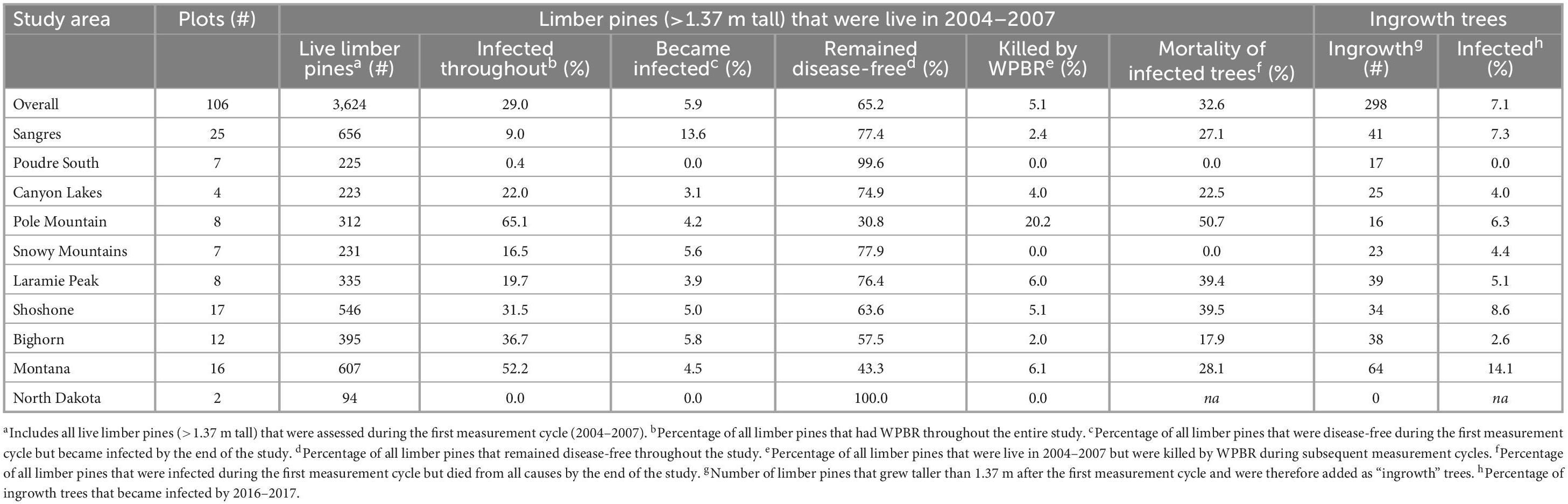
Table 3. Changes in the health and white pine blister status for all live limber pines assessed in the first measurement cycle to the last measurement cycle (2004/2006/2007 to 2016/2017) and white pine blister rust status for trees that grew taller than 1.37 m after the first measurement cycle and therefore were included in the plot as “ingrowth”.
Disease incidence (# live infected trees/# live trees) increased slightly overall from start to end (29.0 to 30.5%, respectively) but not significantly (Table 4). Incidence increased significantly in the Sangre de Cristo Mountains over the study, going from 7.0 to 16.0% (P = 0.006), and declined significantly on Pole Mountain, going from 63.7 to 53.2% (P = 0.0007) (Table 4). Incidence was lowest in Poudre Canyon South (2.3%) and highest on Pole Mountain (53.2%) at the time of the final measurement cycle (no rust detected in North Dakota) (Table 4).
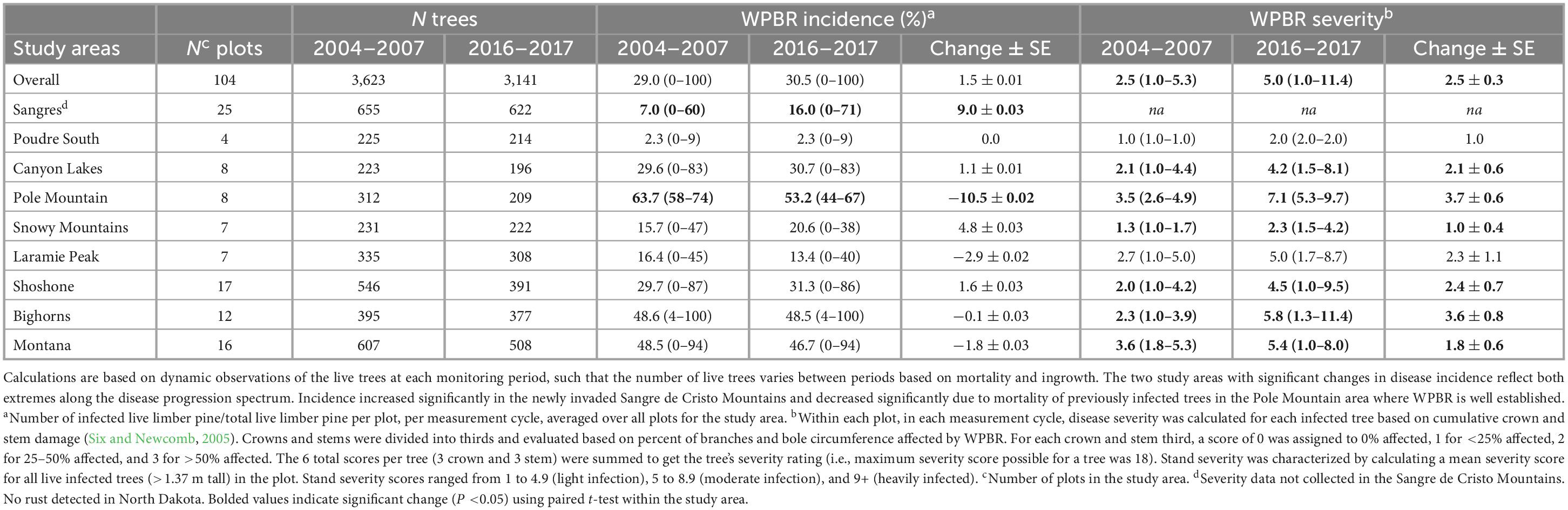
Table 4. Change in white pine blister rust incidence and severity in all live limber pine (>1.37 m tall) assessed for plots at the start (2004–2007) and end (2016–2017) of the study.
Stand density of limber pine (>1.37 m tall) declined by 33% over the study in the Pole Mountain area, where WPBR is well established, and by only 5% in the Sangre de Cristo Mountains, where WPBR has only recently invaded (Figures 5A, B). The decline at Pole Mountain was dominated by mortality of previously diseased trees (51%) while mortality of disease-free trees was largely offset by ingrowth (Table 3 and Figure 5A). The small decline in stand density in the Sangre de Cristo Mountains resulted from a proportionally similar mortality of trees that were diseased and disease-free (Figure 5B). The proportion of the stands composed of diseased trees decreased over time at Pole Mountain (65 to 52% from first to last measurement) and increased in the Sangres (9 to 22%) (Figures 5A, B). When all plots were combined, stand density of limber pine decreased by 14.4% overall with the decrease being experienced by both diseased and disease-free trees (Figure 5C).
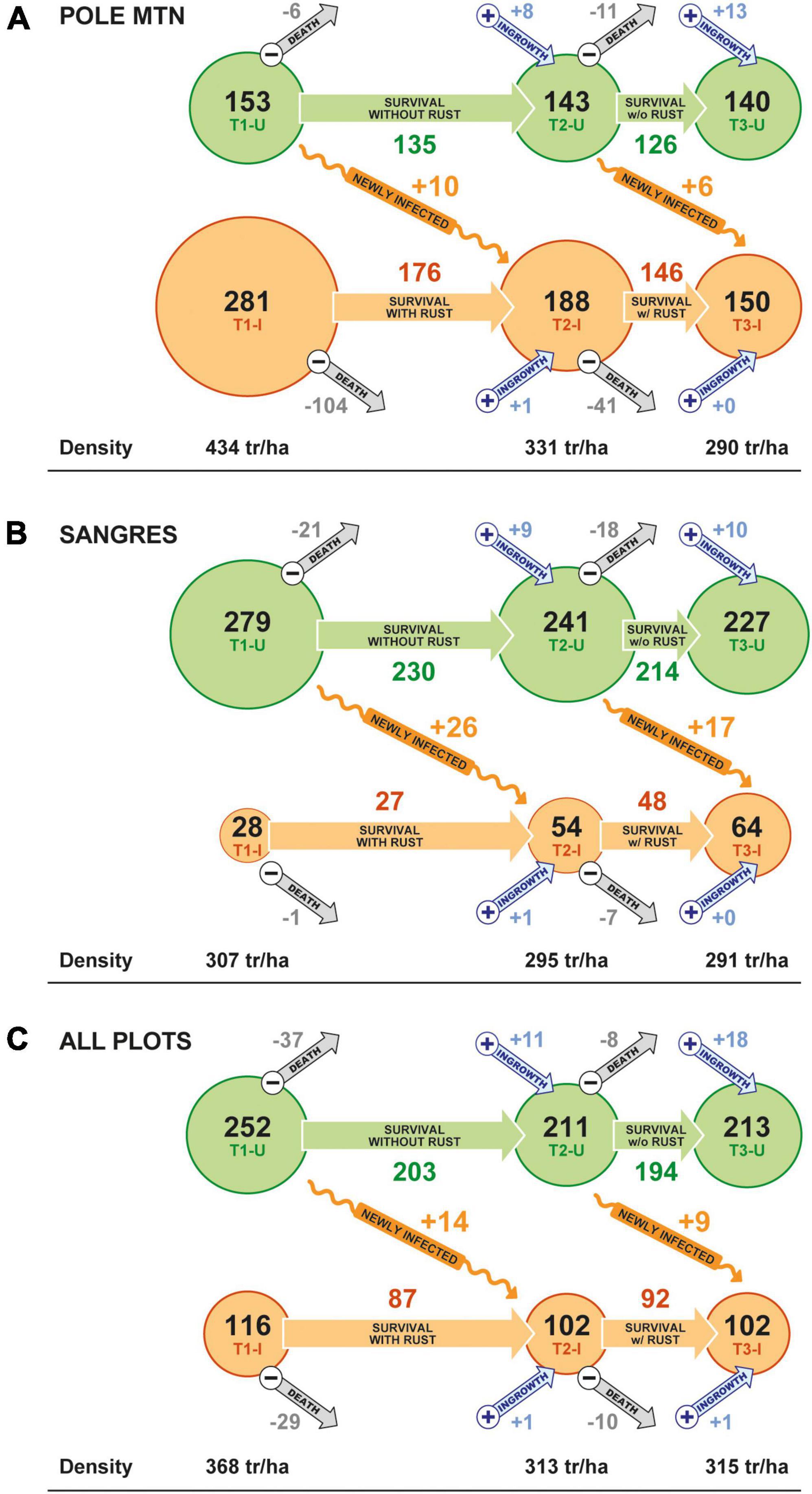
Figure 5. Changes in stand density (trees ha–1) across the three measurement periods (T1, T2, and T3) of disease-free (green circles) and diseased (orange circles) limber pine trees greater than 1.37 m tall in the (A) Pole Mountain area, (B) Sangres area, and (C) for all plots combined. Pole Mountain is an example of where WPBR is well established, and the Sangre de Cristo area is one that has only recently been invaded by C. ribicola. Within each panel, gray arrows depict density loss to mortality (all causes) and blue arrows indicate ingrowth of new trees into the >1.37 m height class. The horizontal distance between T1, T2, and T3 circles generally reflects time between measurement periods. The orange squiggly arrows show the density of trees that transitioned from disease-free to diseased between the two time periods. Average tree density has been rounded to the nearest individual per hectare to simplify the presentation.
While mean dbh of live limber pine overall was 13.0 cm, uninfected trees were 11.7 cm and infected trees 17.8 cm on average during the final measurement cycle, respectively. Most infected trees were less than 30.5 cm dbh and fairly equally distributed in the small and medium diameter classes (Supplementary Table 5b), but most rust-killed trees (85%) were small (<12.7 cm dbh).
Using the rating system established by Six and Newcomb (2005; see section “2.3.3. White pine blister rust assessments”), in 2004–2007, 14 sites (18%) had no rust detected, 62 sites (79%) were lightly infected, 3 sites (4%) were moderately infected, and 0 sites were heavily infected (Supplementary Table 7). However, by 2016–2017, 12 sites (15%) had no infection, 33 sites (42%) had light infection, 30 sites (38%) had moderate infection, and 4 sites (5%) were heavily infected. Overall, WPBR severity increased significantly from 2.5 to 5.0 (+2.5 ± 0.3, P < 0.0001; Table 4) from 2004–2007 to 2016–2017, as did severity in every study area except Poudre South and Laramie Peak (data not collected in Sangre de Cristo Mountains, no WPBR in North Dakota). The range of severity ratings over all plots in 2004–2007 was 1.0–5.3 which significantly increased to 1.0–11.4 in 2016–2017 (P < 0.001), with the highest severity sites (11+ rating) occurring in the Bighorn Mountains. Disease severity was lowest in Poudre South (2.0) and Snowy Mountains (2.3) study areas in 2016–2017.
As others have shown, branch cankers were well distributed throughout tree crowns (Burns et al., 2011; Crump et al., 2011; Jacobi et al., 2016). The average number of branch cankers per infected tree was 3.6 in 2004–2007 and decreased to 2.7 in 2016–2017 (−0.9 ± 0.1, P < 0.0001; Supplementary Table 7). However, the average number of stem cankers per tree increased significantly overall, from 0.7 to 1.0 (+0.3 ± 0.03, P < 0.0001), and in most study areas (Supplementary Table 7). During the first measurement cycle, 13% of infected limber pine (>1.37 m tall) had at least one stem canker in the lower stem third, but this increased to 16% in 2016–2017 (+3.6 ± 0.02%, P = 0.04). Similarly, 38% of infected trees had at least one stem canker at the beginning of the study but this increased to 50% (+11.8 ± 0.03%, P = 0.0004) by the end of the study.
At the time of the first measurement cycle, all subregions except Poudre South had cankers in 60% or more of the first 10 (12-cm) canker size classes. In the final measurement cycle, all subregions except Poudre South (20%) and Canyon Lakes (60%) had cankers in 80% or more of the first 10 (12-cm) canker size classes. Two of the more recently invaded study areas, the Sangre de Cristo and Snowy Mountains, had cankers in 90% or more of the (12-cm) canker size classes as did several study areas where the disease has been present for decades (Pole Mountain, Bighorns, and Shoshone).
3.6. Limber pine regeneration (<1.37 m tall)
The average density of limber pine regeneration in 2011–2013 was 161 stems ha–1 and increased to 181 stems ha–1 in 2016–2017, though this change (20 ± 15 stems ha–1) was not significant (Table 2). In 2011–2013, 4.8% of live stems <1.37 m tall were infected with WPBR which decreased to 4.3% in 2016–2017 though this change was also not significant (−0.4 ± 0.01%, P > 0.5). Of sites monitored for regeneration in 2011–2013 and 2016–2017 (Sangre de Cristo Mountains and North Dakota excluded), 24% had WPBR-infected regeneration in 2011–2013 which decreased to 18% in 2016–2017 though this change was not significant (P = 0.26; data not shown). However, mean WPBR-caused mortality increased significantly over all study areas from the start of the second measurement cycle to the end, going from 1.0% in 2011–2013 to 2.6% in 2016–2017 (+1.6 ± 0.9%, P = 0.03; Table 2). WPBR was the leading cause of death for regeneration (less than 1.37 m tall) during the final measurement cycle; roughly 47% of mortality was directly attributed to WPBR. Significant differences in regeneration density, WPBR incidence, and mortality were not observed in any study area (Table 2). Most sites had ≥20% limber pine species composition, however 6% of sites had no regeneration of any tree species and 24% had no limber pine regeneration observed during the study period. Most sites in the Bighorn Mountains (58%) had no limber pine regeneration throughout the study. Further, the Bighorn Mountains had the highest percentage of sites (25%) with no regeneration of any species followed by the Shoshone NF (18%) and Canyon Lakes (13%).
3.7. Modeling the drivers of white pine blister rust incidence and severity
Over the study period, the broader trends of increasing growing season length and aridity that have been seen in the central and southern Rocky Mountains (McGuire et al., 2012; Dee and Stambaugh, 2019) were also seen here at the plot and study area levels. Within all study areas, the average annual number of days above 5°C (growing degree days) increased by 13 to 53% (10 to 24 days) when comparing the 4-year window preceding the first and last measurement cycles. Similarly, August–September maximum vapor pressure deficit (VPD) increased 5.5 to 13% in six of the nine study areas, (North Dakota not included in this analysis; Supplementary Figure 1).
Variables selected in the final generalized logistic model of WPBR presence at the plot-level include both climatic and site variables. The probability of WPBR being present at a site increased with longer growing season length, if Ribes species were present, and at higher latitude (Table 5 and Figure 6A). The best model to predict tree-level WPBR infection probability included topographic and climatic variables. Probability of infection was greater for trees growing on northern slopes, at higher latitude, and on sites with lower maximum VPD in August and September (Table 5 and Figure 6B).
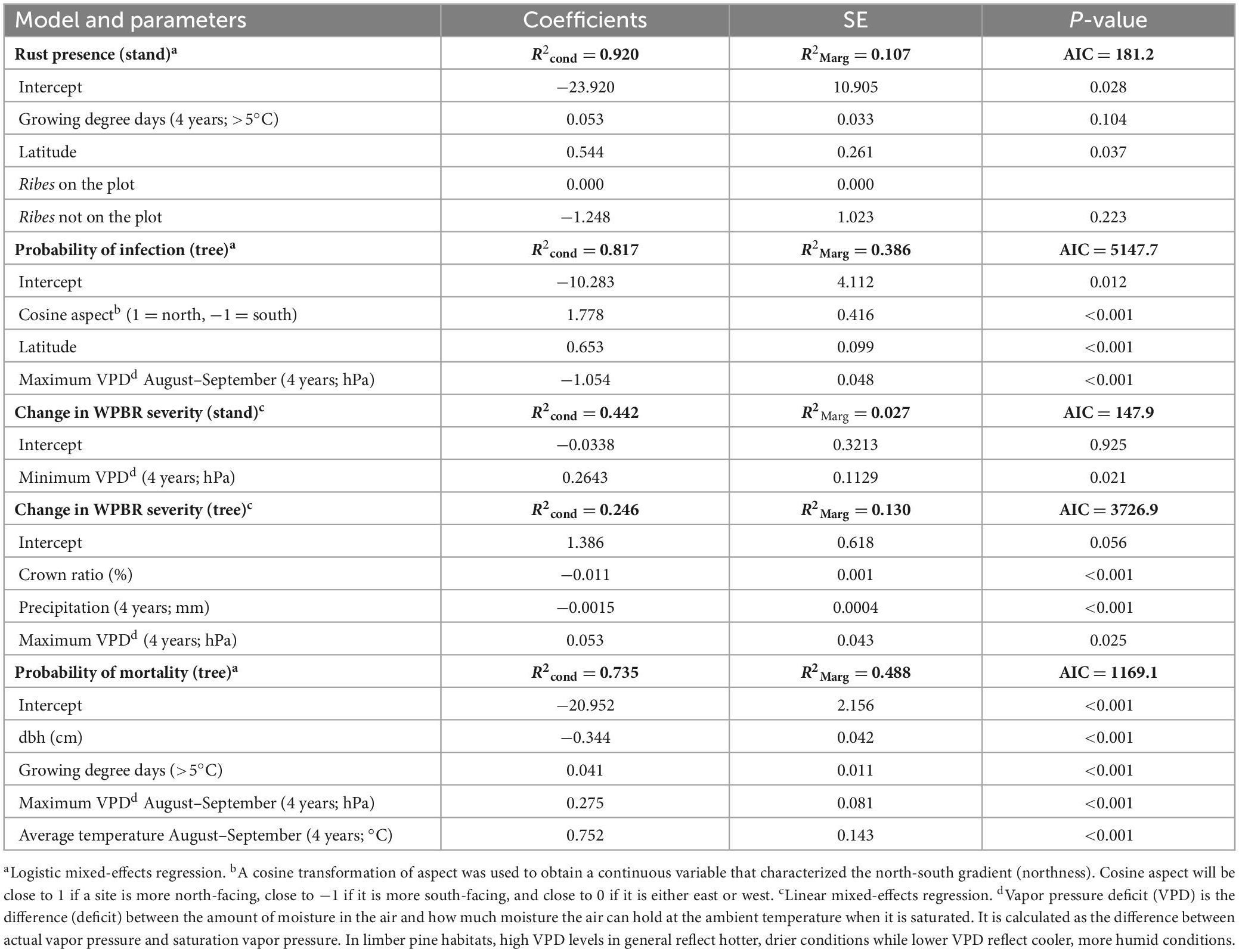
Table 5. Summary of best model subsets from final logistic and linear mixed-effects regressions predicting white pine blister rust presence, infection probability, change in disease severity, and probability of mortality at the tree and stand level.
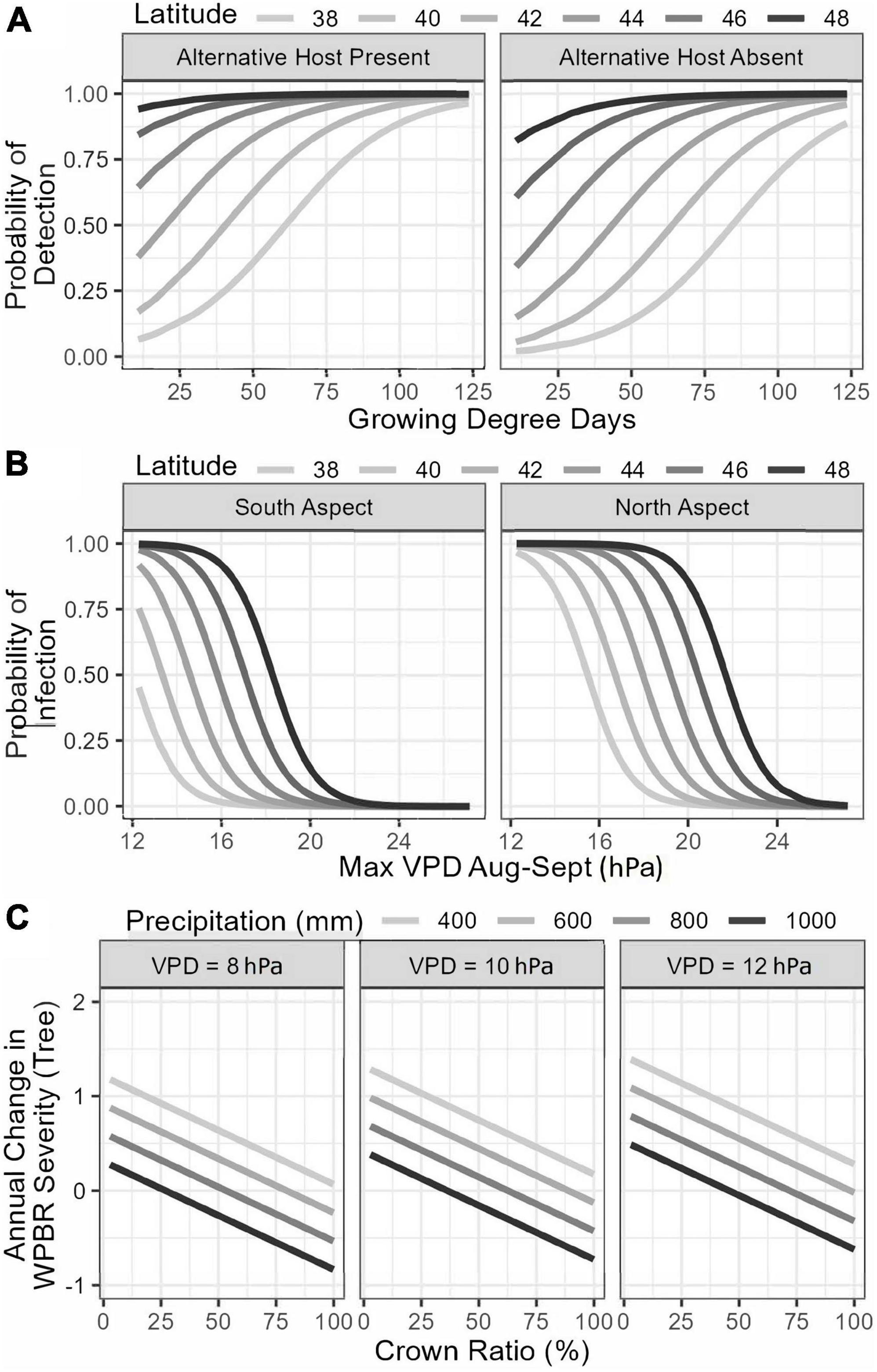
Figure 6. Best subset logistic regression (A,B) and final general linear mixed effects (C) models predicting white pine blister rust presence in a stand (A), tree infection probability (B), and annual change in disease severity (C) based on stand, site, and meteorological variables.
The final generalized linear model explaining changes in plot-level WPBR severity was only significantly linked with a single variable. Plot-level increases in disease severity were greatest on sites with higher minimum VPD (Table 5). At the tree-level, changes in WPBR severity were explained by tree structure and climatic conditions. Infected trees with greater crown ratios had lower rates of annual change in WPBR severity. Additionally, trees on sites with more precipitation had lower annual change rates in WPBR severity, whereas trees at sites with greater maximum VPD had higher rate of change in WPBR severity over time (Table 5 and Figure 6C).
Variables selected in the final general logistic mixed-effects model predicting the probability of rust-caused mortality include tree structure and climatic attributes. The probability of mortality in infected trees was reduced as a tree’s diameter increased. However, infected trees had a higher likelihood of being killed by WPBR on sites with higher maximum VPD in August and September, higher average temperatures in August and September, and as the number of growing degree days increased (Table 5).
4. Discussion
The cumulative impacts associated with WPBR and bark beetle-caused mortality resulted in notable declines of limber pine. Significant increases in the proportion of declining, dying, and dead trees were observed overall and in most study areas. These effects varied at the plot and study area levels depending on whether the disease was at an invading or established phase. By the end of the study, 25% of limber pines were declining or dying, another 25% were standing dead, and live limber pine density and basal area declined significantly. Live limber pine maintained a reverse J-shaped diameter distribution throughout the study indicating that some recruitment is occurring, but mortality (4%/year) greatly outpaced recruitment of ingrowth (∼1%/year). Similar demographic trends have been observed in sugar pine (Pinus lambertiana) in the southern Sierra Nevada (Dudney et al., 2020) and in limber and whitebark pine in southern Alberta (Smith et al., 2013a,b). We also found strong relationships between meteorological factors and WPBR incidence and severity over time indicating long-term vulnerabilities to limber pine health.
While bark beetles and WPBR alone or in combination were significant mortality factors, the timing of these events varied. Most of the observed bark beetle mortality occurred between the first and second measurement cycles (i.e., after 2006/2007 but before 2014) whereas mortality caused by WPBR was chronic, becoming more common after the first measurement cycle and increasing steadily over time. We found similar cumulative mortality caused by bark beetles as Cleaver et al. (2015) in their 2011–2012 survey in a similar geographic area, but in contrast, by the end of our study WPBR was the leading cause of mortality in both trees and regeneration. Further, the proportion of all trees surveyed that were killed by blister rust in our study (5%) was double what Jacobi et al. (2018a) reported from their surveys of limber pine in Wyoming and Colorado between 2004 and 2012 (2.5%).
The incidence of WPBR increased slightly over the course of the study and across all study areas, though not significantly. The lack of significant change is likely due to high mortality of WPBR-infected trees caused by bark beetles and WPBR. However, this trend has shifted over time because new WPBR infections continue to occur consistently while bark beetle mortality has subsided. Like Jacobi et al. (2018a), our analysis demonstrated that infection events occur regularly throughout the relatively dry climates of our study area, both at the disease front and in areas where the pathogen is well established (Kearns et al., 2009). Disease incidence remained the same or increased in study areas where the pathogen is considered “invading” (Sangre de Cristo Mountains, Snowy Mountains, and Poudre South) and remained the same or decreased in study areas where it is considered “established” (e.g., Pole Mountain, Bighorns, and Montana; Jacobi et al., 2018a). Over the study, nearly half of all plots gained more infected trees and 6% of trees that were disease-free during the first measurement cycle and 7% of ingrowth became infected. Still, the current distribution of WPBR is likely underestimated since detection is especially difficult during the early stages of invasion. In the more recently identified outbreak areas, such as the Sangre de Cristo Mountains and Poudre South, blister rust was likely present for at least 10–15 years before it was detected and reported (Burns, 2006; Schoettle et al., 2018). More frequent surveys that coincide with the timing of sporulation on pines are needed to get a more accurate estimate of disease distribution.
Surges in disease severity were evident not only through increased rust-caused mortality but also by substantial increases in stem canker incidence and stand level disease severity ratings. Disease severity increased overall and in every study area where blister rust was detected. The number of stem cankers per infected limber pine also increased significantly from 0.7 to 1.0 stem canker per infected limber pine. This is much higher than the ∼0.2 stem cankers per infected tree that Kearns and Jacobi (2007) found during their 2004 survey in southeast Wyoming and northern Colorado. Our results are in line with a 10-year study examining WPBR on limber pine in Canada that found a similar trend of increasing stem canker incidence over time (Smith et al., 2013a). Stem cankers in the upper and lower stem are both detrimental to limber pine health and survival. Stem cankers in the upper crown reduce cone production since cones occur more frequently on upper crown branches (Steele, 1990), leading to reduced regeneration. Lower stem cankers are much more likely to girdle and kill the entire tree, thus these observed increases suggest higher likelihoods of mortality. In both cases, increased incidence of stem cankers drives decline and mortality, especially in small trees and regeneration. Our data highlights that both sites where rust is well established and those closer to the disease front are facing increasing disease severity which will likely lead to increased mortality.
Variation in WPBR disease incidence across the landscape is likely to continue even as the pathogen becomes well established. Disease incidence is the outcome of complex interactions among environmentally controlled infection probabilities and severity (e.g., this article), geographically variable genetic disease resistance frequencies in pine populations (e.g., Schoettle et al., 2014), pine population size and dynamics (e.g., Field et al., 2012), biotic and abiotic stresses that affect pine health and mortality (e.g., this article; Fettig et al., 2022), and other factors. The ability of recruitment to offset tree mortality and support adaptation to WPBR will determine the sustainability of limber pine populations (Schoettle et al., 2019b,2022a). Consequently, as WPBR becomes naturalized in North America, the surviving populations will likely exhibit diverse spatial and temporal patterns of disease prevalence, as is observed for native forest diseases (Burdon and Thrall, 1999).
We developed models to identify meteorological variables, tree and stand characteristics, and site factors associated with the probability of WPBR infection, rust-caused mortality, and changes in disease severity at the tree and stand level. The probability of tree infection was greater on sites with northern exposure (cooler), higher latitude (longer pathogen presence), and lower maximum August and September VPD (less arid). Unlike what others have reported, tree and stand characteristics were not important predictors of rust presence or incidence in our study (Smith and Hoffman, 2001; Kearns and Jacobi, 2007; Kearns et al., 2014; Cleaver et al., 2015). However, our results align with other studies that found increasing probability of infection where environmental conditions are cooler and more humid, and on sites located in more northern geographic positions (Kearns and Jacobi, 2007; Cleaver et al., 2015). A recent study of limber pine condition along Montana’s Rocky Mountain Front suggests the positive association between infection probability and latitude may not persist over time because new WPBR infections appear to have plateaued (Cleaver et al., 2022). While they reported a small proportion of new infections, they also found no evidence of recent wave years, which may be due to hotter, drier conditions associated with climate change. If western forests experience drought and warmer temperatures in the future as anticipated (McGuire et al., 2012; Dee and Stambaugh, 2019), wave year events may occur less frequently. However, our analysis of canker sizes indicated that infections occurred nearly every year in most areas throughout the study even though the U.S. Rocky Mountains were experiencing persistent drought.
Our study found strong relationships between aridity and WPBR infection probability, disease severity progression, and tree mortality. The probability of WPBR-induced mortality was greater for trees, particularly with smaller diameters, growing on sites with a longer growing season, and higher average temperature and maximum VPD in August and September (more arid), consistent with Dudney et al. (2021). Similarly, we observed greater annual change in WPBR severity for infected trees growing on sites with lower annual precipitation and higher maximum VPD (more arid), suggesting that disease severity and subsequent mortality may increase in the future as climates become warmer and drier. Limber pine stomata are particularly responsive to changes in VPD (Pataki et al., 2000) so at high maximum VPD, stomatal closure would likely restrict infection. Higher minimum VPD levels would suggest sustained conditions of high evaporative demand that could lead to water stress in trees if available soil water was limited (as is likely in August and September in most of the Rockies). Our modeling suggests that sustained late season water stress contributes to accelerated disease progression, resulting in mortality. Other studies have shown that canker diseases induce loss of sapwood hydraulic function thereby reducing a tree’s capacity to survive increased exposure to severe drought conditions (Hossain et al., 2018). Drought can also reduce tree defenses to bark beetles resulting in more successful beetle attacks leading to mortality (e.g., Bentz et al., 2022).
Natural selection against WPBR-susceptible individuals is evident, especially in study areas with high WPBR incidence such as Pole Mountain. Declining density of diseased trees within populations due to WPBR-caused mortality is reducing population size and thus disease incidence. This pattern was also reported for heavily impacted limber pine populations in southern Alberta (Smith et al., 2013a). The relative stability of the density of disease-free trees at Pole Mountain is, in part, a consequence of ingrowth offsetting new tree infections. Genetic resistance to WPBR is likely also a contributing factor as qualitative resistance, associated with a single resistance gene, is present in limber pine in this area (Schoettle et al., 2014). Qualitative resistance causes an immunity response which is conferred by a single dominant resistance gene (an R gene) which curtails disease progression, and therefore visible disease symptoms, in infected trees (Kinloch and Dupper, 2002). Qualitative resistance can also be referred to as complete resistance or major gene resistance (MGR). The resistance gene Cr4 conveys this immunity response in limber pine (Schoettle et al., 2014). An average of 5% of the pre-WPBR invaded limber pine population in the southern Rockies is estimated to have the Cr4 R gene and that frequency can be 13.9% or higher in some stands (Schoettle et al., 2014, 2019a). Therefore, it is likely that many of the remaining disease-free trees at Pole Mountain have the Cr4 resistance allele. If enough resistant trees persist and reproduce, stand density may recover over time (barring other disturbances). This may take many decades since it can take more than 50 years for limber pine to mature and many more to produce a full cone crop (Johnson, 2001). However, if a virulent strain of C. ribicola develops in the area that can overcome Cr4-resistance, there will likely be another surge in disease incidence and a further reduction in stand density.
Quantitative resistance to WPBR also exists in limber pine but the limited available research suggests that its frequency may be very low in limber pine populations (Jacobi et al., 2018b; Schoettle et al., 2022b). In contrast to qualitative resistance, quantitative resistance is conferred by the small contribution of many genes which makes it less likely to be overcome by a simple mutation in the rust and therefore is more durable over time. Quantitative resistance to WPBR suppresses but generally does not prevent disease (King et al., 2010). Individuals show a range of susceptibility, presumably dependent on which and how many genes are inherited that contribute to resistant phenotypes (King et al., 2010). The effectiveness of some of the traits can be reduced by high C. ribicola infection (King et al., 2010; Jacobi et al., 2018b). This in combination with other mortality factors (e.g., fire and bark beetles) can reduce the available quantitative and qualitative resistance traits to WPBR in the population, further increasing the probability of WPBR-caused mortality (Schoettle and Sniezko, 2007; Schoettle et al., 2022a). Furthermore, the frequency of quantitative resistance traits alone may be too low in limber pine to sustain viable populations, especially under high WPBR pressure, without active management (Schoettle et al., 2022a).
Tree mortality by WPBR, i.e., natural selection, has only just begun in the newly invaded study areas, such as the Sangre de Cristo Mountains. Increasing WPBR incidence over time demonstrates that the population contains abundant susceptible individuals on which selection can act. When combining results from all study plots, one could incorrectly infer that WPBR incidence is stable across the U.S. Rockies over the sampling time of this study. However, closer inspection reveals that the forest health condition is not uniform, highlighting the importance of scale over which forest health information is coalesced. The invasion and adaptation process to WPBR is at different stages and proceeding at different rates across the greater U.S. Rockies landscape (Schoettle et al., 2022a).
Regeneration was assessed over time and provides insight into trends regarding changes in limber pine composition and abundance over time. Though most sites surveyed had at least 20% composition of limber pine in the overstory, 24% of sites had no limber pine regeneration and 6% of sites had no regeneration of any species throughout the entire study period. Our study found limber pine regeneration on fewer sites than Cleaver et al. (2017b) in Colorado and Wyoming and Smith et al. (2013a) in Alberta, Canada. While we found increasing limber pine regeneration abundance over the course of the study, it was only present on 50, 55, and 59% of plots at the start, middle, and end, respectively. In contrast, Cleaver et al. (2017b) found limber pine regeneration present on 92% of plots in 2011–2012, but average density was slightly lower overall than what we report here (141 vs. our 181 stems ha–1). Smith et al. (2013a) reported an increase in limber pine regeneration in plots in Alberta, Canada over a 10-year period from 76% in 2003–2004 to 85% in 2009 having at least one limber pine seedling, and density increased from 100 seedling ha–1 to 150 seedling ha–1 in the same time frame. While limited in our study plots, it is possible that limber pine regeneration is present in other forest types of the U.S. Rocky Mountains (Windmuller-Campione and Long, 2016). Goeking and Windmuller-Campione (2021) analyzed a large USDA Forest Service, Forest Inventory and Analysis 10-year dataset and found that limber pine regeneration occurred more abundantly in non-limber pine forest types.
While WPBR incidence remained stable in limber pine regeneration (∼4.5%) over the study, we observed a significant increase (1.0–2.6%, +1.6%) in WPBR-caused morality in regeneration. When considered with our low regeneration rates, increasing mortality of regeneration is concerning because as overstory mortality occurs fewer limber pine seedlings may survive long enough to replace them. While rust incidence levels were similar to Cleaver et al. (2017b; 5.3%), our mortality levels were higher, which one would expect given more time. As observed in Smith et al. (2013a), the short period in which small seedlings may become infected with WPBR, die, and degrade from a site makes determining trends in WPBR infection and mortality difficult and likely underreported. Due to our relatively long remeasurement cycles (∼5 years) seedling infection and mortality was likely greater than what we observed.
It is likely that WPBR and episodic bark beetle outbreaks, the length and severity of which are dependent on many factors including favorable climatic and stand conditions, and proximity to bark beetle populations, will continue to be prominent damage agents in these regions (Jacobi et al., 2018a; Fettig et al., 2022). Therefore, long-term monitoring will be critical to inform and guide conservation and management efforts to retain and restore this ecologically important species as climates change in the U.S. Rocky Mountains. Though there are inherent difficulties in maintaining long-term study plots in remote areas, the data provided by measuring monumented trees over time enables characterizing changes in extent and progression of slow spreading damage agents. Such studies also allow the identification of healthy populations to focus proactive management strategies (Schoettle et al., 2019b,2022a). Likewise, populations with high WPBR-caused mortality may provide putatively resistant seed sources for additional rust-resistance screening and outplanting. Additionally, management efforts to preserve multiple diameter classes can promote stand resilience when faced with future bark beetle episodes and continued WPBR impacts (Schoettle and Sniezko, 2007).
5. Conclusion
Assessing species composition over time, particularly after a significant portion of the limber pine population was killed by the recent bark beetle epidemic and ongoing WPBR infections, allowed for insight on how forest landscapes are being affected by these biotic factors. Live limber pine density and basal area significantly decreased over all plots. Limber pine is considered an early seral species outcompeted in moderate environmental conditions by species such as subalpine fir, Engelmann spruce, and Douglas-fir, but on harsh, rocky, and xeric sites it is considered a climax species (Veblen, 1986; Rebertus et al., 1991; Donnegan and Rebertus, 1999). However, 7% of study sites had no regenerating species of any kind recorded. Coupled with the significant health decline and mortality of limber pine, these numbers are unsettling regarding recruitment and resiliency in these sites in the future.
As WPBR is now a permanent biotic factor of North American ecosystems, evaluating disease spread through the remaining range of white pines and continued monitoring of disease intensification and impacts will be critical for developing effective management strategies that are appropriate for specific forest health conditions to preserve this ecologically important species. Though bark beetle populations have returned to endemic levels, a large proportion of live limber pines that survived are now declining or dying, primarily due to WPBR. These results confirm that WPBR is negatively affecting the health of limber pine populations in the U.S. Rocky Mountains and will continue to do so over time, as suggested by our modeling results. The dramatic increase in severity of WPBR impacts, both at the tree and plot levels, is further evidence of this. With climate models predicting continued warming, there is potential for more frequent and severe bark beetle epidemics in the future, making the identification and preservation of WPBR-resistant limber pines increasingly critical (Schoettle and Sniezko, 2007; Schoettle et al., 2022a).
Data availability statement
The raw data supporting the conclusions of this article will be made available by the authors, without undue reservation.
Author contributions
KB, WJ, AS, and JS conceived of the study. KB and KL collected the field data. All authors contributed to the data analysis and writing, editing, and approving the final manuscript.
Funding
This study was funded by the U.S. Department of Agriculture, Forest Service, Forest Health Protection, Evaluation Monitoring Program to KB and JS (INT-EM-B-16-01).
Acknowledgments
We thank Great Sand Dunes National Park and Preserve (F. Bunch and P. Pineda-Bovin), Rocky Mountain National Park (B. Verhulst and J. Connor), Blackfeet Indian Reservation, Montana DNRC (A. Gannon), and U.S. Department of Agriculture, Forest Service personnel from the Shoshone, Bighorn, Medicine Bow, Roosevelt, San Isabel, and Rio Grande National Forest for logistical support. We especially thank all of those who helped with field work, data entry, and analyses: A. Casper, J. Blodgett, J. Backsen, M. Jackson, G. DeNitto, J. Klutsch, B. Howell, M. Halford, M. Laskowski, R. Beam, J. Hof, E. Janasov, S. Doria, B. Lalande, S. Stratton, B. Stone, C. Holtz, S. Stephens, and D. Shorrock. The contributions of U.S. Government employees to this work were supported in part by their respective agencies.
Conflict of interest
The authors declare that the research was conducted in the absence of any commercial or financial relationships that could be construed as a potential conflict of interest.
Publisher’s note
All claims expressed in this article are solely those of the authors and do not necessarily represent those of their affiliated organizations, or those of the publisher, the editors and the reviewers. Any product that may be evaluated in this article, or claim that may be made by its manufacturer, is not guaranteed or endorsed by the publisher.
Author disclaimer
The findings and conclusions in this publication are those of the authors and should not be construed to represent any official U.S. Department of Agriculture or U.S. Government determination or policy.
Supplementary material
The Supplementary Material for this article can be found online at: https://www.frontiersin.org/articles/10.3389/ffgc.2023.1149456/full#supplementary-material
Footnotes
References
Alfaro, R. I., Campbell, R., Vera, P., Hawkes, B., and Shore, T. (2003). “Dendroecological reconstruction of mountain pine beetle outbreaks in the Chilcotin Plateau of British Columbia,” in Mountain pine beetle symposium: Challenges and solutions, information report BC-X-399, eds T. L. Shore, J. E. Brooks, and J. E. Stone (Kelowna: Natural Resources Canada), 245–256.
Allen, M., Pereira, R. G., Raes, L. S., and Smith, D. (2006). FAO irrigation and drainage paper. Crop evapotranspiration (Guidelines for computing crop water requirements). Paper No. 56. Rome: Food and Agriculture Organization, 46.
Bates, D., Mächler, M., Bolker, B., and Walker, S. (2014). Fitting linear mixed-effects models using lme4. arXiv [Preprint] doi: 10.48550/arXiv.1406.5823
Bentz, B. J., Millar, C. I., Vandygriff, J. C., and Hansen, E. M. (2022). Great Basin bristlecone pine mortality: Causal factors and management implications. For. Ecol. Manag. 509:120099. doi: 10.1016/j.foreco.2022.120099
Blodgett, J. T., and Sullivan, K. F. (2004). First report of white pine blister rust on Rocky Mountain bristlecone pine. Plant Dis. 88, 311–311. doi: 10.1094/PDIS.2004.88.3.311A
Brown, P. M., and Schoettle, A. W. (2008). Fire and stand history in two limber pine (Pinus flexilis) and Rocky Mountain bristlecone pine (Pinus aristata) stands in Colorado. Int. J. Wildland Fire 17, 339–347. doi: 10.1071/WF06159
Burdon, J. J., and Thrall, P. H. (1999). Spatial and temporal patterns in coevolving plant and pathogen associations. Am. Nat. 153, S15–S33. doi: 10.1086/303209
Burns, K. (2006). White pine blister rust in the Sangre de Cristo and wet mountains of southern Colorado, biological evaluation, R2-06-05. Lakewood, CO: US Forest Service Rocky Mountain Regional Office.
Burns, K., Blodgett, J., Jackson, M., Howell, B., Jacobi, W., Schoettle, A., et al. (2011). “Monitoring limber pine health in the Rocky Mountains and North Dakota,” in Proceedings of the future of high-elevation, five-needle white pines in Western North America: High five symposium, proceedings RMRS-P-63, eds R. E. Keane, D. F. Tomback, M. P. Murray, and C. M. Smith (Fort Collins, CO: U.S. Department of Agriculture Forest Service), 47–50.
Cerezke, H. (1995). Egg gallery, brood production, and adult characteristics of mountain pine beetle, Dendroctonus ponderosae Hopkins (Coleoptera: Scolytidae), in three pine hosts. Can. Entomol. 127, 955–965. doi: 10.4039/Ent127955-6
Cleaver, C., Gannon, A., Hanna, D., McKeever, K., Jackson, M., Kramer, A., et al. (2022). “Limber pine condition along Montana’s Rocky Mountain front,” in Forest health monitoring: National status, trends, and analysis 2021. General technical report. SRS-266, eds K. M. Potter and B. L. Conkling (Asheville, NC: U.S. Department of Agriculture Forest Service Southern Research Station), 177–181. doi: 10.2737/SRS-GTR-266-Chap12
Cleaver, C. M., Burns, K. S., and Schoettle, A. W. (2017a). Limber pine and white pine blister rust monitoring and assessment guide for Rocky Mountain National Park. Inter-agency agreement 15-IA-11221633-157. Fort Collins, CO: U.S. Department of Agriculture Forest Service Rocky Mountain Research Station, 28.
Cleaver, C. M., Jacobi, W. R., Burns, K. S., and Means, R. E. (2017b). Limber pine regeneration and white pine blister rust in the central and southern Rocky Mountains. For. Sci. 63, 151–164. doi: 10.5849/forsci.16-052
Cleaver, C. M., Jacobi, W. R., Burns, K. S., and Means, R. E. (2015). Limber pine in the central and southern Rocky Mountains: Stand conditions and interactions with blister rust, mistletoe, and bark beetles. For. Ecol. Manag. 358, 139–153. doi: 10.1016/j.foreco.2015.09.010
Coop, J. D., and Schoettle, A. W. (2009). Regeneration of Rocky Mountain bristlecone pine (Pinus aristata) and limber pine (Pinus flexilis) three decades after stand-replacing fires. For. Ecol. Manag. 257, 893–903. doi: 10.1016/j.foreco.2008.10.034
COSEWIC (2014). COSEWIC assessment and status report on the limber pine Pinus flexilis in Canada. Ottawa: COSEWIC, 49.
Crump, A., Jacobi, W. R., Burns, K. S., and Howell, B. E. (2011). Pruning to manage white pine blister rust in the Southern Rocky Mountains. Research note RMRS-RN-44. Fort Collins, CO: U.S. Department of Agriculture Forest Service Rocky Mountain Research Station, 10. doi: 10.2737/RMRS-RN-44
Dee, J. R., and Stambaugh, M. C. (2019). A new approach towards climate monitoring in Rocky Mountain alpine plant communities: A case study using herb-chronology and Penstemon whippleanus. Arct. Antarct. Alp. Res. 51, 84–95. doi: 10.1080/15230430-2019.1585173
Donnegan, J. A., and Rebertus, A. J. (1999). Rates and mechanisms of subalpine forest succession along an environmental gradient. Ecology 80, 1370–1384. doi: 10.1890/0012-9658(1999)080[1370:RAMOSF]2.0.CO;2
Dudney, J., Willing, C. E., Das, A. J., Latimer, A. M., Nesmith, J. C., and Battles, J. J. (2021). Nonlinear shifts in infectious rust disease due to climate change. Nat. Commun. 12:5326. doi: 10.1038/s41467-021-25182-6
Dudney, J. C., Nesmith, J. C., Cahill, M. C., Cribbs, J. E., Duriscoe, D. M., Das, A. J., et al. (2020). Compounding effects of white pine blister rust, mountain pine beetle, and fire threaten four white pine species. Ecosphere 11:e03263. doi: 10.1002/ecs2.3263
Dunlap, J. (2012). Variability in and environmental correlates to white pine blister rust incidence in five California white pine species. Northwest Sci. 86, 248–263. doi: 10.3955/046.086.0402
Fettig, C. J., Asaro, C., Nowak, J. T., Dodds, K. J., Gandhi, K. J., Moan, J. E., et al. (2022). Trends in bark beetle impacts in North America during a period (2000–2020) of rapid environmental change. J. For. 120, 693–713. doi: 10.1093/jofore/fvac021
Field, S. G., Schoettle, A. W., Klutsch, J. G., Tavener, S. J., and Antolin, M. F. (2012). Demographic projection of high-elevation white pines infected with white pine blister rust: A nonlinear disease model. Ecol. Appl. 22, 166–183. doi: 10.1890/11-0470.1
Furniss, R. L. (1977). Western forest insects. Washington, DC: U.S. Department of Agriculture Forest Service. doi: 10.5962/bhl.title.131875
Geils, B. W., Hummer, K. E., and Hunt, R. S. (2010). White pines, Ribes, and blister rust: A review and synthesis. For. Pathol. 40, 147–185. doi: 10.1111/j.1439-0329.2010.00654.x
Gibson, K. (2003). Mountain pine beetle: Conditions and issues in the western United States, 2003, information report BC-X-399. Victoria: Pacific Forestry Centre, 57–61.
Gibson, K., Skov, K., Kegley, S., Jorgensen, C., Smith, S., and Witcosky, J. (2008). Mountain pine beetle impacts in high-elevation five-needle pines: Current trends and challenges, R1-08-020. Missoula, MT: U.S. Department of Agriculture Forest Service, 1–32.
Goeking, S. A., and Windmuller-Campione, M. A. (2021). Comparative species assessments of five-needle pines throughout the western United States. For. Ecol. Manag. 496:119438.
Hossain, M., Veneklass, E. J., Hardy, G. E. S. J., and Poot, P. (2018). Tree host-pathogen interactions as influenced by drought timing: Linking physiological performance, biochemical defense and disease severity. Tree Physiol. 39, 6–18. doi: 10.1093/treephys/tpy113
Jackson, M., Gannon, A., Kearns, H., and Kendall, K. (2010). Current status of limber pine in Montana. Report R1-10-06. Washington, DC: U.S. Department of Agriculture Forest Service, 14.
Jacobi, W. R., Bovin, P. P., Burns, K. S., Crump, A., and Goodrich, B. A. (2016). Pruning limber pine to reduce impacts from white pine blister rust in the Southern Rocky Mountains. For. Sci. 63, 218–224. doi: 10.5849/forsci.16-011
Jacobi, W. R., Kearns, H. S., Cleaver, C. M., Goodrich, B. A., and Burns, K. S. (2018a). Epidemiology of white pine blister rust on limber pine in Colorado and Wyoming. For. Pathol. 48:e12465. doi: 10.1111/efp.12465
Jacobi, W. R., Kearns, H. S., Kegley, A., Savin, D. P., Danchok, R., and Sniezko, R. A. (2018b). “Comparative look at rust infection and resistance in limber pine (Pinus flexilis) and Rocky Mountain bristlecone pine (P. aristata) following artificial inoculation at three inoculum densities,” in Proceedings of the IUFRO joint conference: Genetics of five-needle pines, rusts of forest trees, and Strobusphere, RMRS-P-76, eds A. W. Schoettle, R. A. Sniezko, and J. T. Kliejunas (Fort Collins, CO: U.S. Department of Agriculture Forest Service), 151–157.
Johnson, K. A. (2001). Pinus flexilis. Fire effects information system. Fort Collins, CO: U.S. Department of Agriculture Forest Service Rocky Mountain Research Station.
Kearns, H., Jacobi, W., Reich, R., Flynn, R., Burns, K., and Geils, B. (2014). Risk of white pine blister rust to limber pine in Colorado and Wyoming, USA. For. Pathol. 44, 21–38. doi: 10.1111/efp.12065
Kearns, H. S., and Jacobi, W. R. (2007). The distribution and incidence of white pine blister rust in central and southeastern Wyoming and northern Colorado. Can. J. For. Res. 37, 462–472. doi: 10.1139/x06-231
Kearns, H. S., Jacobi, W. R., and Geils, B. W. (2009). A method for estimating white pine blister rust canker age on limber pine in the central Rocky Mountains. For. Pathol. 39, 177–191.
King, J., David, A., Noshad, D., and Smith, J. (2010). A review of genetic approaches to the management of blister rust in white pines. For. Pathol. 40, 292–313. doi: 10.1111/j.1439-0329.2010.00659.x
Kinloch, B. B. Jr., and Dupper, D. E. (2002). Genetic specificity in the white pine-blister rust pathosystem. Phytopathology 92, 278–280. doi: 10.1094/PHYTO.2002.92.3.278
Kliejunas, J., and Dunlap, J. (2007). “Status of whitebark pine and other high-elevation five-needle pines with emphasis on Pacific coast ecosystems; what are the issues and concerns? Perspective from California,” in Proceedings of the conference: Whitebark pine: A Pacific coast perspective, eds E. M. Goheen and R. A. Sniezko (Princeton, NJ: Citeseer).
Klutsch, J. G., Goodrich, B. A., and Schoettle, A. W. (2011). “Limber pine forests on the leading edge of white pine blister rust distribution in northern Colorado,” in Proceedings of the future of high-elevation, five-needle white pines in Western North America: High five symposium, proceedings RMRS-P-63, eds R. E. Keane, D. F. Tomback, M. P. Murray, and C. M. Smith (Fort Collins, CO: U.S. Department of Agriculture Forest Service), 222–225.
Klutsch, J. G., Negron, J. F., Costello, S. L., Rhoades, C. C., West, D. R., Popp, J., et al. (2009). Stand characteristics and downed woody debris accumulations associated with a mountain pine beetle (Dendroctonus ponderosae Hopkins) outbreak in Colorado. For. Ecol. Manag. 258, 641–649. doi: 10.1016/j.foreco.2009.04.034
Krebill, R. (1964). Blister rust found on limber pine in northern Wasatch Mountains. Plant Dis. Rep. 50:532.
Krist, F. Jr., Ellenwood, J., Woods, M., McMahan, A., Cowardin, J., Ryerson, D., et al. (2014). National insect and disease forest risk assessment: 2013-2027, FHTET-14-01. Washington, DC: U.S. Department of Agriculture.
Kuznetsova, A., Brockhoff, P. B., and Christensen, R. H. (2017). lmerTest package: Tests in linear mixed effects models. J. Stat. Softw. 82, 1–26. doi: 10.18637/jss.v082.i13
Lachmund, H. G. (1933). Mode of entrance and periods in the life cycle of Cronartium ribicola on Pinus monticola. J. Agric. Res. 47, 791–805.
Langor, D. W. (1989). Host effects on the phenology, development, and mortality of field populations of the mountain pine beetle, Dendroctonus ponderosae Hopkins (Coleoptera: Scolytidae). Can. Entomol. 121, 149–157. doi: 10.4039/Ent121149-2
Larson, E. R. (2011). Influences of the biophysical environment on blister rust and mountain pine beetle, and their interactions, in whitebark pine forests. J. Biogeogr. 38, 453–470. doi: 10.1111/j.1365-2699.2010.02430.x
Logan, J. A., and Powell, J. A. (2001). Ghost forests, global warming, and the mountain pine beetle (Coleoptera: Scolytidae). Am. Entomol. 47, 160–173. doi: 10.1093/ae/47.3.160
Lüdecke, D., Ben-Shachar, M. S., Patil, I., and Makowski, D. (2020). Extracting, computing and exploring the parameters of statistical models using R. J. Open Source Softw. 5:2445. doi: 10.21105/joss.02445
Malone, S. L., Schoettle, A. W., and Coop, J. D. (2018). The future of subalpine forests in the Southern Rocky Mountains: Trajectories for Pinus aristata genetic lineages. PLoS One 13:e0193481. doi: 10.1371/journal.pone.0193481
Maloney, P. E. (2011). Incidence and distribution of white pine blister rust in the high-elevation forests of California. For. Pathol. 41, 308–316.
McDonald, G. I., and Hoff, R. J. (2001). “Blister rust: An introduced plague,” in Whitebark pine communities: Ecology and restoration, eds D. F. Tomback, S. F. Arno, and R. E. Keane (Washington, DC: Island Press), 193–220.
McGuire, C. R., Nufio, C. R., Bowers, M. D., and Guralnick, R. P. (2012). Elevation-dependent temperature trends in the Rocky Mountain Front Range: Changes over a 56- and 20-year record. PLoS One 7:e44370. doi: 10.1371/journal.pone.0044370
Millar, C. I., Charlet, D. A., Westfall, R. D., King, J. C., Delany, D. L., Flint, A. L., et al. (2018). Do low-elevation ravines provide climate refugia for subalpine limber pine (Pinus flexilis) in the Great Basin, USA? Can. J. For. Res. 48, 663–671. doi: 10.1139/cjfr-2017-0374
Monahan, W. B., Cook, T., Melton, F., Connor, J., and Bobowski, B. (2013). Forecasting distributional responses of limber pine to climate change at management-relevant scales in Rocky Mountain National Park. PLoS One 8:e83163. doi: 10.1371/journal.pone.0083163
O’Hara, K. L. (2002). The historical development of uneven-aged silviculture in North America. Forestry 75, 339–346. doi: 10.1093/forestry/75.4.339
Pataki, D. E., Oren, R., and Smith, W. K. (2000). Sap flux of co-occurring species in a western subalpine forest during seasonal soil drought. Ecology 81, 2557–2566. doi: 10.1890/0012-9658(2000)081[2557:SFOCOS]2.0.CO;2
Rebertus, A., Burns, B., and Veblen, T. (1991). Stand dynamics of Pinus flexilis-dominated subalpine forests in the Colorado front range. J. Veg. Sci. 2, 445–458. doi: 10.2307/3236026
Rehfeldt, G. E., Ferguson, D. E., and Crookston, N. L. (2008). Quantifying the abundance of co-occurring conifers along Inland Northwest (USA) climate gradients. Ecology 89, 2127–2139. doi: 10.1890/06-2013.1
Schoettle, A. W. (2004). “Ecological roles of five-needle pine in Colorado: Potential consequences of their loss,” in Breeding and genetic resources of five-needle pines: Growth, adaptability and pest resistance, proceedings RMRS-P-32, eds R. A. Sniezko, S. Samman, S. Schlarbaum, and H. Kriebel (Fort Collins, CO: US Department of Agriculture Forest Service), 124–135.
Schoettle, A. W., Burns, K. S., Cleaver, C. M., and Connor, J. J. (2019a). Proactive limber pine conservation strategy for the Greater Rocky Mountain National Park Area. General Technical Report RMRS-GTR-379. Fort Collins, CO: U.S. Department of Agriculture Forest Service Rocky Mountain Research Station, 81. doi: 10.2737/RMRS-GTR-379
Schoettle, A. W., Jacobi, W. R., Waring, K. M., and Burns, K. S. (2019b). Regeneration for resilience framework to support regeneration decisions for species with populations at risk of extirpation by white pine blister rust. New For. 50, 89–114. doi: 10.1007/s11056-018-9679-8
Schoettle, A. W., Burns, K. S., McKinney, S. T., Krakowski, J., Waring, K. M., Tomback, D. F., et al. (2022a). Integrating forest health conditions and species adaptive capacities to infer future trajectories of the high elevation five-needle white pines. For. Ecol. Manag. 521:120389. doi: 10.1016/j.foreco.2022.120389
Schoettle, A. W., Kegley, A., Sniezko, R. A., Burns, K. S., Vogler, D., Bovin, P. P., et al. (2022b). “Preparing for invasion: Rust resistance in limber, Great Basin bristlecone, and Rocky Mountain bristlecone pines,” in Proceedings of the research and management of high-elevation five-needle pines in Western North America: Second high-five conference, eds M. P. Murray, C. M. Smith, S. T. McKinney, and P. L. Achuff (Arcata, CA: The Press at Cal Poly Humboldt), 77–80.
Schoettle, A. W., Goodrich, B., Klutsch, J., and Burns, K. S. (2018). “White pine blister rust confirmed on limber pine (Pinus flexilis) in Rocky Mountain National Park,” in Proceedings of the IUFRO joint conference: Genetics of five-needle pines, rusts of forest trees, and strobusphere, Proc. RMRS-P-76, eds A. W. Schoettle, R. A. Sniezko, and J. T. Kliejunas (Fort Collins, CO: U.S. Department of Agriculture Forest Service Rocky Mountain Research Station), 201–204.
Schoettle, A. W., and Sniezko, R. A. (2007). Proactive intervention to sustain high-elevation pine ecosystems threatened by white pine blister rust. J. For. Res. 12, 327–336. doi: 10.1007/s10310-007-0024-x
Schoettle, A. W., Sniezko, R. A., Kegley, A., and Burns, K. S. (2014). White pine blister rust resistance in limber pine: Evidence for a major gene. Phytopathology 104, 163–173. doi: 10.1094/PHYTO-04-13-0092-R
Schwandt, J. W. (2006). Whitebark pine in peril: A case for restoration. Report R1-06-28. Missoula, MT: U.S. Department of Agriculture Forest Service.
Shanahan, E., Legg, K., Daley, R., Irvine, K., Wilmoth, S., and Jackson, J. (2019). Monitoring five-needle pine on bureau of land management lands in Wyoming summary report for 2013, 2014, 2016, 2017. Natural resource report NPS/GRYN/NRR—2019/1931, 102. Bozeman, MT: Northern Rocky Mountain Science Center.
Six, D. L., and Newcomb, M. (2005). A rapid system for rating white pine blister rust incidence, severity, and within-tree distribution in whitebark pine. Northwest Sci. 79, 189–195.
Smith, C. M., Langor, D. W., Myrholm, C., Weber, J., Gillies, C., and Stuart-Smith, J. (2013a). Changes in white pine blister rust infection and mortality in limber pine over time. Can. J. For. Res. 43, 919–928. doi: 10.1139/cjfr-2013-0072
Smith, C. M., Shepherd, B., Gillies, C., and Stuart-Smith, J. (2013b). Changes in blister rust infection and mortality in whitebark pine over time. Can. J. For. Res. 43, 90–96. doi: 10.1139/cjfr-2012-0127
Smith, J. P., and Hoffman, J. T. (2001). Site and stand characteristics related to white pine blister rust in high-elevation forests of southern Idaho and western Wyoming. West. N. Am. Nat. 61, 409–416.
Steele, R. (1990). Pinus flexilis James limber pine, Agriculture Handbook 654. Silvics N. Am. 1, 348–354.
Taylor, S. W., Carroll, A. L., Alfaro, R. I., and Safranyik, L. (2006). “Forest, climate and mountain pine beetle outbreak dynamics in western Canada,” in The mountain pine beetle. A synthesis of biology, management and impacts on lodgepole pine, eds L. Safranyik and B. Wilson (Victoria: Natural Resources Canada - Canadian Forest Service-Pacific Forestry Centre), 67–94.
R Core Team (2015). R: A language and environment for statistical computing. Vienna: R Foundation for Statistical Computing.
Thornton, P., Thornton, M., Mayer, B., Wei, Y., Devarakonda, R., Vose, R., et al. (2016). Daymet: Daily surface weather data on a 1-km grid for North America, version 3. Oak Ridge, TN: ORNL DAAC.
Tomback, D. F., Keane, R. E., McCaughey, W. W., and Smith, C. (2005). Methods for surveying and monitoring whitebark pine for blister rust infection and damage. Missoula, MT: Whitebark Pine Ecosystem Foundation.
Veblen, T. T. (1986) Age and size structure of subalpine forests in the Colorado Front Range. Bulletin of the Torrey Botanical Club, pp. 225–240.
Vogler, D. R., Geils, B. W., and Coats, K. (2017a). First report of the white pine blister rust fungus, Cronartium ribicola, infecting Ribes inerme in north-central Utah. Plant Dis. 101, 386–386. doi: 10.1094/PDIS-09-16-1253-PDN
Vogler, D. R., Maloney, P. E., Burt, T., and Snelling, J. (2017b). First report of the white pine blister rust fungus, Cronartium ribicola, infecting Pinus flexilis on Pine Mountain, Humboldt National Forest, Elko County, Northeastern Nevada. Plant Dis. 101, 839–839. doi: 10.1094/PDIS-10-16-1502-PDN
Vorster, A. G., Evangelista, P. H., Stohlgren, T. J., Kumar, S., Rhoades, C. C., Hubbard, R. M., et al. (2017). Severity of a mountain pine beetle outbreak across a range of stand conditions in Fraser Experimental Forest, Colorado, United States. For. Ecol. Manag. 389, 116–126. doi: 10.1002/eap.2059
Windmuller-Campione, M. A., and Long, J. N. (2016). Limber pine (Pinus flexilis James), a flexible generalist of forest communities in the intermountain west. PLoS One 11:e0160324. doi: 10.1371/journal.pone.0160324
Keywords: white pine blister rust, limber pine, mountain pine beetle, climate change, stand condition assessment, long-term monitoring, forest health management, vapor pressure deficit
Citation: Burns KS, Tinkham WT, Leddy KA, Schoettle AW, Jacobi WR and Stewart JE (2023) Interactions between white pine blister rust, bark beetles, and climate over time indicate vulnerabilities to limber pine health. Front. For. Glob. Change 6:1149456. doi: 10.3389/ffgc.2023.1149456
Received: 22 January 2023; Accepted: 07 March 2023;
Published: 03 April 2023.
Edited by:
Isabel Alvarez Munck, Forest Health Protection, Forest Service (USDA), United StatesReviewed by:
Cameron Ducayet McIntire, Forest Service (USDA), United StatesDavid Thoma, United States Department of the Interior, United States
Copyright © 2023 Burns, Tinkham, Leddy, Schoettle, Jacobi and Stewart. This is an open-access article distributed under the terms of the Creative Commons Attribution License (CC BY). The use, distribution or reproduction in other forums is permitted, provided the original author(s) and the copyright owner(s) are credited and that the original publication in this journal is cited, in accordance with accepted academic practice. No use, distribution or reproduction is permitted which does not comply with these terms.
*Correspondence: Kelly S. Burns, kelly.burns@usda.gov; Jane E. Stewart, jane.stewart@colostate.edu